Coastdat.eu
Final Draft
of the original manuscript:
Yang, X.; Zhao, L.; Almasy, L.; Garamus, V.M.; Zou, A.;
Willumeit, R.; Fan, S.:
Preparation and characterization of 4-dedimethylamino
sancycline (CMT-3) loaded nanostructured lipid carrier
(CMT-3/NLC) formulations
In: International Journal of Pharmaceutics (2013) Elsevier
DOI: 10.1016/j.ijpharm.2013.04.021
Preparation and characterization of 4-dedimethylamino sancycline
(CMT-3) loaded nanostructured lipid carrier (CMT-3/NLC)
Xiaomin Yang1a Lin Zhao1c Laszlo Almasyd Vasil M. Garamusb Aihua Zoua Regine Willumeitb Saijun Fanc
State Key Laboratory of Bioreactor Engineering and Institute of Applied Chemistry, East China University of
Science and Technology, Shanghai 200237, PR China,
Helmholtz-Zentrum Geesthacht: Centre for Materials and Coast Research, Institute of Materials Research,
Max-Planck-Str. 1, D-21502 Geesthacht, Germany
School of Radiation Medicine and Protection, Soochow University Medical College, Suzhou 215123, PR
Research Institute for Solid State Physics and Optics, HAS, Konkoly-Thege Miklós út 29-33, 1525 Budapest,
* To whom correspondence should be addressed. Tel/Fax.: +86-21-64252063.
E-mail:
[email protected].
East China University of Science and Technology.
Helmholtz-Zentrum Geesthacht.
Soochow University Medical College
Abstract
Chemical modified tetracyclines (CMTs) have been reported to have strong
inhibition ability on proliferation and invasion of various cancers, but its application is
restricted for its poor water solubility. In present study, hydrophilic CMT-3 loaded
nanostructured lipid carrier (CMT/NLC) was produced by high pressure
homogenization (HPH). The physical properties of CMT/NLC were characterized by
dynamic light scattering (DLS), high efficiency liquid chromatography (HPLC),
atomic force microscopy (AFM), Scan electron microscopy (SEM), Small-Angle
Neutron Scattering (SANS), small angle and wide angle X-ray scattering (SAXS and
XRD) The lipids and surfactants ingredients, as well as drug/lipids (m/m) were
investigated for stable and sustained NLC formulations. In vitro cytotoxicity of
CMT/NLC was evaluated by MTT assay against HeLa cells. The diameter of
CMT/NLC increased from 153.1±3.0 nm to maximum of 168.5±2.0 nm after 30 days
of storage while the entrapment efficiency kept constant at >90 %. CMT/NLC
demonstrated burst-sustained release profile in release mediums with different pH,
and the 3-dimension structure of CMT/NLC was suspected to attribute this release
pattern. The results of cell uptaking and location indicated that NLC could arrive at
cytoplasm and NLC makes CMT-3 entering HeLa cells easier. All results showed that
NLC was important to CMT-3 application not only in lab research but also in clinical
field. To the best of our knowledge this is the first report on CMT/NLC.
Key words: Nanostructured lipid carriers; CMT-3; SANS&SAXS; XRD; in-vitro
release; cytotoxicity
1. Introduction
Chemically modified tetracyclines (CMTs) are analogs of tetracycline (Fig 1),
which is used clinically as an antibiotic agent. Golub and co-workers first investigated
and described that CMTs lack antimicrobial properties but still inhibit matrix
metalloproteinases (MMPs) (Golub et al., 1991). Since then, numerous in vivo and in
vitro studies have demonstrated that CMT-3, also known as COL-3, is the most
promising anti-tumor molecular compared to other CMTs (Lokeshwar et al., 2002).
CMT-3 has been shown to have strong inhibition ability on proliferation and invasion of
various cancer, such as E-10, MDA-MB-468 human breast cancer cells, COLO 205
colon carcinoma cells, and DU-145, TSU-PR1, and Dunning MAT LyLu human
prostate cancer cells (Gu et al., 2001; Lokeshwa, 1999; Lokeshwa et al., 1998;
Lokeshwar et al, 2001; Lokeshwar et al, 2002; Meng et al., 2000). The anti-metastatic
effect of CMT-3 also has been assessed in the bone metastasis model of MAT LyLu
human prostate cancer cells in rats and the lung metastasis model of C8161 human
melanoma cells in SCID mice (Seftor et al., 1998; Selzer et al., 1999). Though CMT-3
had significant effect on inhibiting tumor metastasis and had several potential
advantages over conventional tetracyclines, the adverse effects included nausea,
vomiting, liver function tests abnormalities, diarrhea, mucositis, leukopenia, and
thrombocytopenia were observed in clinical trials (Syed et al., 2004). The main reason
may attribute to CMT-3's hydrophobic and lipophilic ability, which make it
concentrate in high fatty tissue and produce toxic effects. Because of the poor
water-solubility, the drug is hard to be absorbed into human blood and interstitial fluid,
and it is difficult to be transported into the target human body or abnormal tissue and
organ effectively. On the other hand, because CMT-3 can't be dissolved totally in
saline, the injection manner of giving drug may be forbidden in clinical usage. So, the
improvement of CMT-3 water-solubility has great importance in its clinic application.
Fig 1. The structures of doxycycline (upper) and CMT-3 (lower).
Over the past several decades, much interest has focused on the design of more
efficient drug delivery systems to address problems such as low drug solubility. The
particulate delivery systems address a number of characteristics including appropriate
size distribution, high drug loading, prolonged release, low cellular cytotoxicity and
cellular targeting. The nanostructured lipid carrier was first developed by Prof. Rainer
H. Müller during the late 1990s (Eliana et al., 2010). NLC is composed of mixture of
solid and liquid lipid compounds such as triacylglycerols, fatty acids, steroids, and oil
(Rainer et al., 2007). NLC is attractive for its combination of advantages of many
other drug carriers (solid lipid nanoparticles, polymeric nanoparticles, liposomes and
emulsions) (Rainer et al., 2004). NLC can be produced on a large scale using lipids
and surfactants that are already accepted, and long-term stability NLC formulations
have been reported for various applications (Khalil et al., 2011; Medha et al., 2009).
NLC can enhance lipophilic drug solubility by virtue of its lipids core and aqueous
shell. Enhances solubility is significant because a great number of drug candidates are
poorly soluble. Also, the solid matrix provides NLC with sustained release properties,
as the degradation or erosion of the lipid matrix releases the incorporated drugs from
NLC. Finally, the nanosize of NLC increases its therapeutic efficacy and reduces
toxicity. In this study, CMT-3 was incorporated into a NLC made up of biodegradable
and biocompatible fatty acids and triacylglycerols. The aim of this study was to
design long-term stable water soluble CMT/NLC formulations with proper size, high
drug loading and sustained release profiles. Cellular uptake and cellular location were
investigated using rhodamine B as a probe for these high efficiency antitumor
formulations. To our knowledge, this is the first repot on CMT/NLC, and there has no
evidence showing CMT-3 loaded other nanoformulations so far. The results in this
study imply that this CMT-3 loaded nanocarrier may significantly improve the
effectiveness of CMT-3 in clinical applications.
2. Materials and methods
2.1 Materials
4-dedimethylamino sancycline (CMT-3, CollaGenex Pharmaceuticals Inc,
Newtown, Pennsylvania); Steric acid (SA, LingFeng Chemical Reagent Co. Ltd,
China ); monoglyceride (MGE, Aladdin Chemical Reagent Co. Ltd, China ); oleic
acid (OA, Aladdin Chemical Reagent Co. Ltd, China); capric/caprylic triglycerides
(MCT, Aladdin Chemical Reagent Co. Ltd, China); Cremophor EL(Aladdin Chemical
Reagent Co. Ltd, China); Pluronic F68 (Adamas Reagent Co. Ltd, China); freshly
prepared double distilled and ultra purified water; trehalose (Aladdin Chemical
Reagent Co. Ltd, China); 3-(4,5-dimethylthiazol-2-yl)-2,5-diphenyltetrazolium
bromide (MTT, Sigma-Aldrich, MO, USA), D2O (Sigma, Germany).
2.2. Preparation, particle sizes and size distribution of NLCs
NLC were prepared by high pressure homogenizer (HPH). In brief, aqueous
phase which consisted of double distilled water and one or two surfactants
mixture(1/1, m/m) and oil phases (lipid mixtures, including SA, MGE, OA and MCT)
were separately prepared. Desired oil phase were maintained at 70 °C to prevent the
recrystallization of lipids during the process, then CMT-3 was added to the oil phase
which was stirred until completely dissolved. The same temperature aqueous phase
was added to the oil phase with intense stirring (10000 rmp for 1 min; Turrax T25,
Fluko, Germany). These dispersions were processed through an HPH (ATS
Engineering, Canada) with five homogenization cycles at 600 bar. The
nanodispersions were cooled overnight at room temperature to obtain the
nanostructure lipid carrier.
All samples were lyophilized for long stability. Appropriate amounts of trehalose
(3% w/v in water) were used to dilute the NLC dispersions. The samples were frozen
at -78 °C for 10 h before being lyophilized for 36 h. The freeze-dried powders were
rehydrated with phosphate buffer solution (PBS, pH 7.4) for later experiments.
The mean particle size and polydispersity of NLCs were measured by dynamic
light scattering at 25 °C using Nano-ZS90 system (Malvern Instruments Ltd., UK)
with a measurement angle of 90 °.
2.3. Entrapment efficiency (EE) and drug loading (DL)
To determine the amount of CMT-3, methanol was added to CMT/NLC
formulations to destroy the NLC structure and dissolve the CMT-3 that was released.
The content of CMT-3 was determined by HPLC (Wufeng, China) using the following
experiment conditions: Diamond C18 column (150 nm×4.6 nm i.d, pore size 5µm; A
yi te, China), the mobile phase MeOH: H2O (0.5% TFA, v/v) = 30:70 (v/v), flow rate:
1 mL/min, and wavelength: 360 nm. The calibration curve of CMT-3 concentration
against peak area was C=8.86793*10-7A+0.00248 (R2=0.9999). All the experiments
were conducted at room temperature (25 °C). The drug loading (DL%) and
entrapment efficiency (EE%) were calculated by the following formulas:
DL% = the weight of CMT-3 encapsulated in the NLC / the total weight of CMT/NLC
EE% = the calculated DL / the theoretical DL ×100.
2.4. NLC morphology study
The surface morphology of NLCs was examined by Nanofirst-3100 AFM
(Suzhou Hai Zi Si Nanotechnology Ltd, China). Samples for AFM were prepared by
placing a drop of freshly prepared unloaded blank-NLC and CMT/NLC on the mica
sheet and drying by spin coating.
Scan electron microscopy (SEM, Auriga 40, Zeiss, Germany) was used to study
the internal structures of blank-NLC and CMT/NLC. Before scanning, the samples
were placed on the conductive double-sided sticky tape and then coated with gold in
an argron atmosphere.
2.5. Small-Angle Neutron Scattering
SANS measurements were performed on the Yellow Submarine instrument at the
BNC in Budapest (Hungary) (Rosta L, 2002). The overall q-range was from 0.03 to 1
nm-1. The samples were filled in Hellma quartz cells of 2 mm path length and placed
in a thermostated holder kept at 20.0±0.5 °C. The raw scattering patterns were
corrected for sample transmission, room background, and sample cell scattering. The
2-dimensional scattering patterns were azimuthally averaged, converted to an absolute
scale and corrected for detector efficiency dividing by the incoherent scattering
spectra of 1 mm thick pure water. The scattering from PBS buffer prepared in D2O
was subtracted as the background. Fourier Transformation (IFT) was applied in this
study to analyse the scattering pattern.
2.6.
Small-Angle X-Ray Scattering
The SAXS measurements were performed at laboratory SAXS instrument
(Nanostar, Bruker AXS GmbH, Karlsruhe, Germany). Instrument includes IμS
micro-focus X-ray source with power of 30 W (used wavelength Cu Kα) and
VÅNTEC-2000 detector (14×14 cm2 and 2048×2048 pixels). Sample to detector
distance is 108.3 cm and accessible q range from 0.1 to 2.3 nm-1.
2.7. Wide-Angle X-ray powder diffraction
The crystalline structure of CMT-3, unloaded blank-NLC and CMT/NLC were
investigated by D/MAX 2550 VB/PC X-ray diffractometry (Rigaku, Japan). Aqueous
blank-NLC and CMT/NLC were lyophilized before the XRD measurement.
Diffractograms were obtained from the initial angle 2 θ = 10 ° to the final angle 60 °
with a Cu Kα radiation source. The obtained data were collected with a step width of
0.02 ° and a count time of 1 s.
2.8. In vitro release of CMT-3
The in vitro release of CMT-3 from CMT/NLC was conducted by dialysis bag
diffusion (Xu et al., 2009). 5 mL fresh prepared CMT/NLC solution (100 μg/ml) was
placed into a pre-swelled dialysis bag with 7 KDa MW cutoff. The dialysis bag was
incubated in release medium (PBS, pH 7.4 VS pH 5.5; 20 mL) with 0.5 % of Tween
80 to enhance the solubility of released free CMT-3 and to avoid its aggregation at
37 ℃ under horizontal shaking. At predetermined time points, the dialysis bag was
taken out and placed into a new container containing fresh release medium (20 mL).
The content of CMT-3 in release medium was determined by HPLC as described by
section 2.3. The release rates of CMT-3 were expressed as the mass of CMT-3 in
release medium divided by time.
2.9. Cell morphology
HeLa cells were seeded in 6-well plate after 0.25 % trypsin digestion at a density
of 3×106 per well. After 12 h, cells were exposed to 20 μM CMT-3, blank-NLC and
CMT/NLC for 6 h. The cell morphology was captured by digital camera (Olympus).
2.10. Cell culture
The human cervical cancer cell line HeLa was purchased from American Type
Culture Collection (Manassas, VA, USA). The cells were seeded into cell culture
dishes containing DMEM supplemented with 10 % new calf serum,
L-glutamine (5
mmol/L), non-essential amino acids (5 mmol/L), penicillin (100 U/mL), and
streptomycin (100 U/mL) (Invitrogen, Carlsbad, CA, USA), at 37 °C in a humidified
5% CO2 atmosphere.
2.11. In vitro cellular cytotoxicity assays
Cell viability was measured by 3-(4,5-dimethylthiazol-2-yl)-2,5-dipheny
ltetrazoliumbromide (MTT) assay. HeLa cells were plated in 96-well plates with 100
μL medium at a density of 8×103 per well. After 12 h, cells were exposed to various
concentrations of CMT-3, blank-NLC and CMT/NLC for 24 and 48 h. MTT solution
was directly added to the media in each well, with a final concentration of 0.5 mg/mL
and incubated for 4 h at 37 °C. The formazan crystals were solubilized with 150 μL
DMSO. The absorbance was measured using an enzyme-linked immunosorbent assay
reader at 570 nm, with the absorbance at 630 nm as the background correction. The
effect on cell proliferation was expressed as the percent cell viability. Untreated cells
were taken as 100 % viable.
2.12. Cellular uptake of NLC formulations
Rhodamine B (RB, Sigma-Aldrich, MO, USA) was used as probe to study the
uptake and location of NLC in Hela cells. RB was encapsulated in NLC, and the free
RB was removed via dialysis bag (MW 7000). HeLa cells were seeded in Lab Tek
chamber slides (Nunc, Wiesbaden, Germany) at the density of 2×104 and incubated
for 24 h. The real-time subcellular localization was determined using Cell'R Live Cell
Station (Olympus Company, Inc). The images were captured every 30 second and last
for 15 min. RB/NLC was added at equivalent rhodamine B immediately after the first
3. Results and discussion
3.1. Preparation, particle sizes and distribution of NLCs
In this study, monoglyceride and stearic acid (solid lipids) and oleic acid and
capric/caprylic triglycerides (liquid lipids) were selected for their high ability to
dissolve CMT-3. The non-toxic, non-ionic surfactants, Cremophor EL and Pluronic
F68 were used to increase the stability of NLC.
Many methods have been reported for NLC preparation, including hot and cool
homogenization (Liu et al., 2010), microemulsion (Soheila et al., 2010),
ultrasonication (Wang et al., 2009), solvent emulsion and phase inversion. Among
these, hot homogenization and solvent emulsion have been widely applied, and given
the point that CMT-3 is easily dissolved in organic solvent, high pressure
homogenization (HPH) was used to prepare CMT/NLC for high entrapment
Table 1. Effects of lipid ingredients on mean particle size of blank-NLC.
Mean particle PDI*
Lmix-1 1113 557 415 415 190±3.2 0.30
Lmix-2 1113 557 593 237 150±5.0 0.14
Lmix-3 1074 596 553 277 148±1.9 0.15
Lmix-4 1074 596 593 237 138±4.2 0.20
SA (stearic acid) is a saturated fatty acid with an 18 carbon-chain length, and a
highly lipophilic character. It is often used as solid lipid for the production of NLC.
The selection of MCT as liquid lipid was for its thermodynamic stability, and high
solubility for many drugs (Patricia et al., 2011). In addition, its less ordered structure
is better for imperfect NLC, which is critical for high EE and DL. In fact, as indicated
in Table.1, formulas (Lmix-2 VS Lmix-1, and Lmix-4 VS Lmix-3) with high MCT
showed smaller sizes and more uniform dispersion, while the incorporation of OA
increased the particle size and PDI. This was mainly because the melting point of OA
is low and therefore OA increases the mobility of the internal lipids and fluidity of the
surfactant layer (Radheshyam and Kamla, 2011). Consequently, the ratios between
lipids were fixed as MGE/SA = 2/1, and CMT/OA = 2/1 (m/m).
Table 2. Effects of the concentration of surfactants on mean particle size and stability.
Total surfactant
Mean particle size (nm)
concentration (%)
The studies of surfactants are of great importance for the development of stable
NLC formulas. In addition, while moving through the vasculature, NLC interacted
with various components of blood. The hydrophilic of NLC avoid protein adsorption
on the surface and hence induce delayed immune clearance (Yoo. et al., 2011).
Cremophor EL is FDA approved, and already clinically used for intravenous injection.
In addition, F68 was chosen for its long PEG chains, and PEG chains are known for
providing a stealth character to nanocarriers and slow their elimination from the
bloodstream during intravenous injection (Delmas et al., 2010).
According to pre-experiments (surface tension studies, data are not shown) the
ratio between Cremophor EL and F68 was fixed as 1:1. The total surfactant
concentration was also studied. As can be seen from Table 2, the concentration of
surfactants had only slight effects on mean particle size, whereas increasing
concentrations of surfactant had significant influence on the stability of the dispersion.
This could be explained by the fact that high amount of surfactant make the oil phase
disperse more readily into aqueous phase. Also, the new lipid surface presented during
the HPH process was covered by high concentrations of surfactant, thereby providing
higher homogenization efficiency. Too much surfactant, however, lowered the
stability of NLC. Many factors may have contributed to this phenomenon. For
example, the long PEG chains of F68 can interact with each other by hydrogen
bonding, and surfactants may form large size micelles.
3.2. Entrapment efficiency and drug loading
Not only the stability, but also entrapment efficiency and drug loading are vital
for clinical application of CMT/NLC. In section 3.1, the type and concentration of
lipid ingredients and surfactants were investigated for stable and small size NLC
formulas, in this section the effects of the ratio between drug and lipids on entrapment
efficiency and drug loading was studied.
Table 3. The effects of lipids and CMT-3 content on mean particle size. Values are
mean ± SD (n=3)
Experiments Lipid CMT-3
Mean particle size Mean particle size
38.5 140.0±3.2 145.7±4.9
60.5 145.8±4.9 154.5±5.0
100.0 145.2±5.0 200.0±3.5
100.0 153.1±3.0 168.5±2.0
200.0 142.4±5.1 200.0±4.5
Fig 2. The effects of lipids and CMT-3 concentration on entrapment efficiency and
drug loading. *The labels of samples are the same as in Table. 3. Values are mean ±
The details of CMT/NLC formulas were shown in Table 3, and the mean particle
sizes were measured during 30 days. It was clear that the particle sizes of all formulas
were <200 nm during the storage period. Fig 2 illustrates that the amount of lipids
and CMT-3, and the storage time, had direct relationships with the entrapment
efficiency and drug loading. For the first day after preparation, the entrapment
efficiency and drug loading values of these CMT/NLC formulations (experiments 1 –
5) were in the range of 90 - 96 % and 4.2 - 10.6 %, respectively. It can be concluded
from the data for experiments 1, 2 and 3, that higher drug/lipid ratios didn't increase
the entrapment efficiency. When the amount of CMT-3 is close to its saturation
solubility in the lipid phase, cooling the nanoparticles leads to supersaturation of
CMT-3 in the liquid lipids and consequently to CMT-3 precipitation prior to lipid
precipitation, and therefore to lower entrapment efficiency (Sylvia and Rainer., 2004).
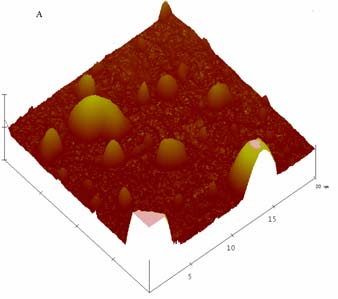
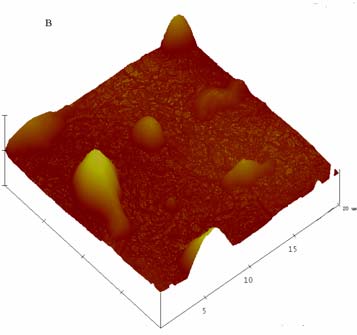
The entrapment efficiency of experiment 4 was higher than that of experiment 3; this
can be explained by the fact that high amounts of lipids increase the solubility of
CMT-3. According to the results from Table 3 and Fig 2, the experiment 4 was chosen
for further studied.
3.3. NLC morphology study
Given that the shape of NLC may affect important biological processes,
including biodistribution and cellular uptake, in drug delivery application
(Venkateraman et al., 2011), AFM was used to investigate the non-hydrated state of
NLCs. It can be seen from Fig 3 that both blank-NLC and CMT/NLC had irregular
morphology with smooth surface. Many factors may induce irregular morphology, for
example, i) the powerful mechanical force and shearing force during preparation, ii)
liquid lipids increase the mobility of lipid phase. But larger particles may result from
the NLC aggregation during spin coating.
Fig 3. AFM images of blank-NLC (A), and CMT/NLC (B).
Fig 4 demonstrated the SEM results of unloaded NLC and CMT loaded NLC
after freeze-drying. Both the blank-NLC and CMT/NLC were located in the bulk and
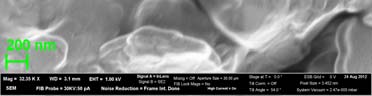
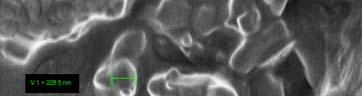
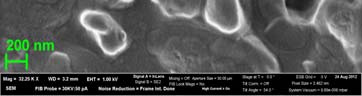
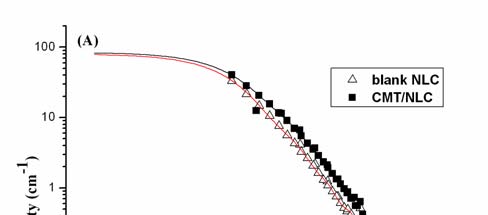
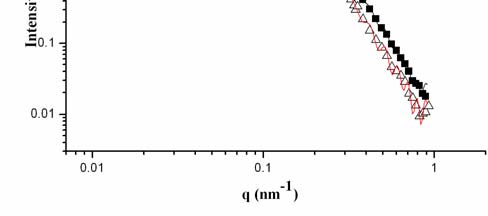
grid structure formed by trehalose. The diameters of from SEM were larger than those
measured by DLS because of the coating before measurement. In addition, the shape
of unloaded NLC was almost spherical, but CMT/NLC was elongated. It was reported
that the elongated, flexible core-shell structures have demonstrated unique
visco-elastic and rheological properties (Ezrahi et. al., 2007; Dreiss, 2007) and the
importance of elongated particles in drug delivery applications has been realized with
the advent of pioneering works of Discher's lab (Geng et. al., 2007; Geng and Discher,
Fig 4. The SEM photographs of blank-NLC (A) and CMT loaded NLC (B).
3.4. Small-Angle Neutron Scattering
Fig 5. SANS spectra of NLC before and after loaded with CMT-3 in PBS (A); P(r)
function obtained from the corresponding scattering curves in A.
SANS was used to study the effect of CMT-3 on the NLC structure. Indirect
fourier transformation (IFT) method was applied in this study. This
model-independent approach needs only minor additional (model) information on the
possible aggregate structure (Glatter., 1977). The experimental data and the fitted
curve coincide very well for both blank NLC and CMT/NLC for all q ranges (Fig
The possible shape and diameter can be obtained from the pair distance
distribution p(r) function (Fig 5B). Blank NLC displayed almost spherical structure
(maximum of p(r) function is located near the middle of maximal size), while after
adding CMT-3 the maximum of p(r) moves to smaller r and it could be interpreted
that the shape became elongated. In fact, this result is agreement with that of SEM.
The particle size (mean diameter) obtained from Fig 5B was significantly smaller than
the data got from DLS. There were two reasons for this disagreement: i) due to
limited qmin SANS data point only on low limit of maximal size of aggregate, ii) DLS
observes hydrated size of particles (particles plus hydrated water) and SANS points to
It is important to obtain the direct information about the structure change from the
large q part of SANS measurements. At a q range, an evidence for fractals in the
submicrometer and nanometer scale can be conveniently derived from small-angle
scattering based on well-known dimensional analysis (Schmidt, 1995). The power law
of the scattering intensity I(q) can be described as I(q) q-α. This exponent indicates the
microscopic structure of scatter can be understood as mass fractals or surface fractals.
When the angular coefficient of the log I(q) versus log q plot is determined, its
relationship with the dimensions of mass and surface fractal, Dv and Ds is α=2×Dv-Ds.
In this study, the α values for blank NLC and CMT/NLC were 3.55 and 3.7,
respectively. It means that such particles have a dense core and rough surface, the core
has a Euclidean dimension, Dv=3, whereas the surface obey a relation Ds=6-α.
Decreasing of value of surface fractal dimension from 2.45 to 2.3 by adding drug to
NLC points on changes of surface of NLC which become smoother. It was in
qualitative agreement with SEM.
3.5. Small-Angle X-Ray Scattering
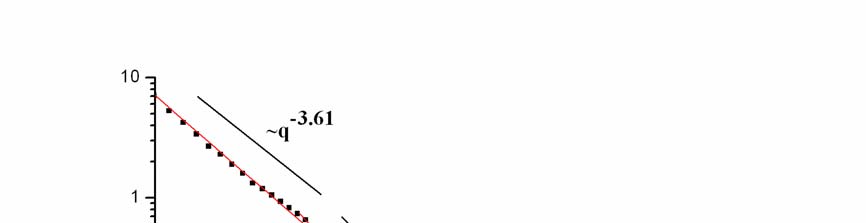
Fig 6. SAXS curve for film freeze-dried blank-NLC and CMT/NLC at 25 °C.
The three-dimensional structures of freeze-dried CMT/NLC and blank NLC were
studied by SAXS. The evaluation of the X-ray spectra was got as reported before
(Seydel et al., 1989; Mariani et al., 1993). There are two peaks (Fig 6, q = 1.31 nm-1,
1.61 nm-1), which means that CMT/NLC is cubic in 3-dimensional structures
(Brandenburg et al., 1998). In fact, blank NLC had the same structures, thus the
adding of CMT-3 did not change the 3-dimensional structures. Low q part of SAXS
has been analyzed by dimensional analysis similar to SANS data obtained for solution
of NLC. Slope of I(q) vs q points to surface fractal structure and corresponds to
surface fractal dimension 2.39 similar to NLC in solution. For blank NLC, the slope
varied from 3.71 to 3.88 at q=0.48 nm-1(curve is not shown), which was coincided
with the rough surface that got from SANS. In the case of CMT/NLC we have also
observed crossover from slope of 3.61 to 4 (smooth surface) at q=0.43 nm-1 which
points on size of primary smooth aggregates forming fractal cluster around 2 nm.
3.6. Wide-Angle X-ray powder diffraction
Fig 7. X-ray diffraction analysis of CMT-3 formulations: X-ray powder
diffractograms of CMT-3 (A), freeze-dried unloaded blank-NLC (B) and freeze-dried
XRD was used to study changes of the microstructure in NLC. XRD analysis
makes it possible to assess the length of the long and short spacing of the lipid lattice.
In Fig 7, the intensities of several diffraction peaks characteristic of CMT-3 reduced in
freeze-dried CMT/NLC, and the diffraction intensity of CMT/NLC was clearly
stronger than that of unloaded blank-NLC. This phenomenon could be attributed to
crystal changes. Many factors can result in changes of crystal structure, for example,
the amount and state of CMT-3 in NLC (Veerawat et al., 2008). Also, the differences
in intensity between blank-NLC and CMT/NLC may be due to the less ordered
microstructure of blank-NLC in comparison to that of CMT/NLC (Lopes et al., 2012).
Thus, adding CMT-3 to imperfect NLC increases crystallinity of CMT/NLC.
3.7. In vitro release of CMT-3
The release experiment was conducted under sink conditions and the dynamic
dialysis was used to separate the CMT-3 that released from CMT/NLC. Fig 8
demonstrated the influence of pH on release profiles of CMT/NLC and release rate of
CMT-3 from CMT/NLC in PBS (0.5 % of Tween 80 in PBS, pH 7.4). It was
obviously that about 58 % CMT-3 released from NLC in PBS (pH 5.6), while for PBS
(pH 7.4) there was about 68 % CMT-3 released from NLC. Thus acidity seemed good
for prolonged release, and CMT-3 had burst release in both medium. But in the
following hours, CMT-3 showed prolonged release profiles. As for release rate (Fig
8B), the release rate of CMT-3 in PBS (pH 7.4) decreased sharply in the first 3 hours,
but after the first 3 hours, the release rate was almost constant (0.02 mg/h) in the
following 45 hours. Thus in both release medium, CMT/NLC exhibited a
burst-prolonged release profile. The solid matrix of NLC and location of CMT-3 in
NLC might attribute to this release pattern. It was reported that the drug can be
incorporated between fatty acid chains, between lipid layers or in imperfections
(Sylvia and Rainer., 2004). During the cooling process, solid lipids (SA and MGE)
rapidly solidified to form solid lipid core for their high melting points, and the rest
liquid lipids distributed randomly around solid lipid core. As liquid lipids had higher
solubility of drug, large amount of drug were loaded in outer lipid layer. In
vitro-release experiment, in the burst release stage, CMT-3 that loaded in shell
released easily and rapidly, while in the sustained release stage, the CMT-3 that
loaded in solid lipid core released by matrix erosion and degradation of lipid
components of NLC, which resulted in prolonged release manner. In addition, the
cubic structure of CMT/NLC protected CMT-3 from leaking from NLC, Other factors
contributing to the fast release are the large surface area, high diffusion coefficient of
nanoparticles, the low viscosity, and short diffusion coefficient of CMT-3 and
surfactant concentration (Zhigaltsev et al., 2010).
Time (hour)
Time (hour)
Fig 8. The in-vitro release study. Cumulative release of CMT-3 in different release
mediums: pH7.4 (A), and pH5.6 (B). Values are mean ± SD (n=3).
3.8. In vitro cellular cytotoxicity assays
In order to know the biological activity of CMT-3 loaded nanoparticles
(CMT/NLC), the cellular cytotoxicity was evaluated by MTT assay. As Fig 9 shows,
CMT-3 exhibits great inhibition effect on Hela cells growth in the given concentration.
For example, the viability of HeLa cells treated with 20 μM CMT-3 for 24 h or 48 h
was 70.9±7.5 % and 61.1±4.4 %, respectively. When treated with 20 μM CMT-3 for
24h, CMT/NLC had more inhibitory ability than CMT-3 (t=3.02, P<0.05, Fig 9A).
Compared to CMT-3, the inhibition effects of CMT/NLC was also remarkably greater
over concentration ranging from 2 to 20 μM when treated for 48 h (t=6.00, 4.40, 4.49,
25.55, P<0.01, Fig 9B), which means the sustained release properties of CMT/NLC
for its cubic structure. It should be pointed out that blank-NLC did not have any
obvious cytotoxicity on HeLa cells, even in high concentration and 48 h exposures.
Fig 9. In vitro cytotoxicity of CMT-3 and CMT/NLC against HeLa cells for 24 h (A)
or 48 h (B). Cell viability is expressed as the percentage of untreated controls. Data
are given as mean±SD (n=6). *p < 0.05 compared with CMT-3.
3.9. Cell morphology
In order to compare the morphology changes of HeLa cells treated with CMT
and CMT/NLC, we used the microscope and digital camera to observe the differences
between the groups. 20 μM CMT-3 exhibits no obvious effect to HeLa cells'
morphology for 6 h (Fig 10B), and the same phenomenon was observed when cells
were treated with blank-NLC (Fig 10C), the volume of which was the same as
CMT/NLC. But, CMT/NLC make cells' morphology changes a lot. As Fig 10D shows,
cell condensation and fragmentation as well as cell shrinkage were observed. The
results indicated that CMT/NLC was easier in leading to cells' toxicity compared to
CMT-3 at the same concentration.
Fig 10. HeLa cells were treated with different medium for 6 h: A. control, without any
treatment; B. 20 μM CMT-3; C. blank-NLC (same volume with CMT/NLC); D.
CMT/NLC (CMT concentration is 20 μM). Cells were captured by digital camera
3.10. Cellular uptake of NLC formulations
We observed the changes of HeLa cells' morphology after treated with RB/NLC.
After HeLa cells were exposed to RB/NLC for 2 h (Fig 11 row 1B shows), it was
clearly that some white pellets appeared around the cells, it was assumed to be
RB/NLC. The control group without CMT/NLC treatment (Fig 11 row 1A shows) has
no this phenomenon.
In order to observe the intracellular distribution of NLC, Cell'R Live Cell Station
was used to capture Hela cells after treatment with RB/NLC every 30 second. As time
went on, the intensity of fluorescence was increasing in cytoplasm in HeLa cell. As
shown in Fig 11 rows 2 - 3, the fluorescence of rhodamine B became obvious at 9 min
when treated with RB/NLC. These demonstrated that RB/NLC can enter into cell's
cytoplasm quickly. In fact, it is realized that many therapeutics (e.g. anti-cancer drugs,
photosensitizers and anti-oxidants) and biotherapeutics (e.g. peptide and protein drugs,
DNA and siRNA) have to be delivered and released into the cellular compartments
such as the cytoplasm or cell nucleus, in order to exert therapeutic effects (Torchilin.,
2006; Nori et al., 2005).
Fig 11. R1(A): control, without RB-NLC treatment; R1(B): HeLa cells were treated
with RB-NLC for 2 h; R2, 3: Red fluorescence from Rhodamine B, where the Hela
cells incubated with RB/NLC at the time of 0, 3, 6, 9,12, 15 minutes, respectively.
All images were captured by Cell'R Live Cell Station (×60).
4. Conclusion
A system of the NLC, i.e. nanostructured lipid carrier, was successfully
developed in this study for controlled and sustainable delivery of anticancer drugs
with CMT-3 as a model drug. The ratios between lipids, drug and surfactants and lipid
types, were studied for their effect on long term stability and uniformity of
nanoparticles size distribution. The NLC particle size (diameter) was about 150 nm
during the 30 days observation period with about 90% encapsulation efficiencies.
SANS showed that CMT/NLC owns smoother surface than blank NLC. And the
shapes of CMT/NLC become elongated, which coincided to SEM result. The
3-dimensional structures of CMT/NLC and blank NLC were cubic according to the
results of SAXS, which was suspected to attribute the burst-sustained release model of
CMT/NLC. The in vitro cellular cytotoxicity assay (MTT) data suggested that NLC
was not cytotoxic to HeLa cells under test conditions, but that CMT/NLC was more
cytotoxic than CMT at the same drug concentration. The increased toxicity of
CMT/NLC was attributed to the increased solubility of CMT-3, a hydrophobic
anticancer drug, in the CMT/NLC formulation. More time exposures resulted in
higher cellular cytotoxicity was agreement with the sustained release properties of
CMT/NLC. Moreover, the possible location of NLC at cytoplasm should also be
favorable to the cytotoxicity improvement of CMT-3. Of course, the exploration of
nano-material carrier to fit CMTs still has a lot of work to do, which will be our next
further research plan.
We would like to express our gratitude to Prof. Leonard I Wiebe for thoughtful
discussions. A. Z. gratefully acknowledges the support of this work by the Alexander
von Humboldt Foundation. We gratefully acknowledge the support of this work by
Chinese National Natural Science Foundation (201003047) and Fundamental
Research Funds for the Central Universities (WK1014024). SANS measurements
have been performed under the support of the European Commission (Grant
agreement N 226507-NMI3). Laszlo Almasy is acknowledged for help during SANS
measurements. Daniel Laipple is acknowledged for help during SEM measurements.
References
Brandenburg, K., Richter, W., Koch, M.H.J., Meyer, H.W., Seydel, U., 1998.
Characterization of the nonlamellar cubic and HⅡ structures of lipid A from
Salmonella enterica serovar Minnesota by X-ray diffraction and freeze-fracture
electron microscopy. Chem.Phys.Lipids. 91, 53-69.
Delmas, T., Couffin, A.C., Bayle, P.A., Crecy, F., Neumann, E., Vinet, F., Bardet, M.,
Bibette, J., Texier, I., 2011. Preparation and characterization of highly stable lipid
nanoparticles with amorphous core of tuneable viscosity. Colloid Interface Sci. 360,
Dreiss, C. A., 2007. Wormlike micelles: Where do we stand? Recent developments,
linear rheology and scattering to techniques. Soft Matter. 956-970.
Eliana, B.S., Rainer, M.H., 2010. Lipid Nanoparticles: Effect on Bioavailability and
Pharmacokinetic Changes. Drug delivery. 115-134.
Ezrahi, S., Tuval, E., Aserin, A., 2006. Properties, main applications and perspectives
of worm micelles. Adv. Colloid Interface Sci. 77-102.
Geng. Y., Discher. D. E., 2005. Hydrolytic degradation of poly(rthylene
oxide)-block-poly(caprolactone) worm micelles. J. Am. Chem. Soc.12780-12781.
Geng. Y., Dalhaimer. P., Cai. S., Tsai. R., Tewari. M., Minko. T., Discher. D.E., 2007.
Shape effects of filaments versus spherical particles in flow and drug delivery. Nat,
Nanotechnol. 249-255.
Glatter. O., 1977. A new method for the evaluation of small-angle scattering data. J.
Appl. Cryst. 10, 415-421.
Golub, L.M., Ramamurthy, N.S., McNamara, T.F., Greenwald, R.A., Rifkin, B.R.,
1991. Tetracyclines inhibit connective tissue breakdown: new therapeutic
implications for an old family of drugs. Crit Rev Oral Biol Med. 2, 297-321.
Gu, Y., Lee, H.M., Roemer, E.J., Musacchia, L., Golub, L.M., Simon, S.R., 2001.
Inhibition of tumor cell invasiveness by chemically modified tetracyclines. Curr
Med Chem. 8, 261–270.
Ji, J.G., Wu, D.J., Liu, L., Chen, J.D., Xu, Y., 2012. Preparation, characterization, and
in vitro release of folic acid-conjugated chitosan nanoparticles loaded with
methotrexate for targeted delivery. Polym. Bull. 68, 1707-1720.
Khalil, M., Ranjita, S., Sven, G., Cecilia, A., Rainer, M.H., 2011. Lipid nanocarrier for
dermal delivery of lutein: Prepartion, characterization, stability and performance.
Int J Pharm. 414, 267-275.
Patricia, S., Samantha, C.P., Eliana, B.S., Maria, H.A.S., 2011. Polymorphism,
crystallinity and hydrophilic-lipophilic balance of stearic acid and stearic
acid-capric/caprylic triglyceride matrices for production of stable nanoparticles.
Colloid and Surface B: Biointerfaces. 86, 125-130.
Lokeshwar, B.L., Selzer, M.G., Zhu, B.Q., Block, N.L., Golub, L.M., 2002. Inhibition
of cell proliferation, invasion, tumor growth and metastasis by an oral
non-antimicrobial tetracycline analog (COL-3) in a metastatic prostate cancer
model. Int J Cancer. 98, 297-309.
Lokeshwar, B.L., 1999. MMP inhibition in prostate cancer. Ann N Y Acad Sci. 878,
Lokeshwar, B.L., Houston-Clark, H.L., Selzer, M.G., Block, N.L., Golub, L.M., 1998.
Potential application of a chemically modifiednon-antimicrobial tetracycline
(CMT-3) against metastatic prostate cancer. Adv Dent Res. 12, 97–102.
Lokeshwar, B.L., Escatel, E., Zhu, B., 2001. Cytotoxic activity and inhibition of
tumor cell invasion by derivatives of a chemically modified tetracycline CMT-3
(COL-3). Curr Med Chem. 8, 271–279.
Lokeshwar, B.L., Selzer, M.G., Zhu, B.Q., Block, N.L., Golub, L.M., 2002. Inhibition
of cell proliferation, invasion, tumor growth and metastasis by an oral
non-antimicrobial tetracycline analog (COL-3) in a metastatic prostate cancer
model. Int J Cancer.98, 297–309.
Lopes, R., Eleuterio, C.V., Goncalves, L.M.D., Cruz, M.E.M., Almeida, A.J., 2012.
Lipid nanoparticles containing oryzalin for the treatment of leishmaniasis. Eur J
Pharm Sci. 45, 442-450.
Liu, Y., Wang, P.F., Sun, C., Feng, N.P., Zhou, W.X., Yang, Y., Tan, R., Chen, Z.Q.,
Wu, S., Zhao, J.H., 2010. Wheat grem agglutinin-grafted lipid nanoparticles:
Preparation and in vitro evaluation of the association with Caco-2 monolayers. Int J
Pharm. 397, 155-163.
Mariani, P., Luzzati, V., Delacroix, H., 1993. Cubic phases of lipid-containing
systems. Structure analysis and biological implications. J. Mol. Biol. 204, 165-189.
Medha, D.J., Rainer, M.H., 2009. Lipid nanoparticles for parenteral delivery of
actives. Eur J Pharm Biopharm. 71, 161-172.
Meng, Q., Xu, J., Goldberg, I.D., Rosen, E.M., Greenwald, R.A., Fan, S., 2000.
Influence of chemically modified tetracyclines on proliferation, invasion and
migration properties of MDA-MB-468 human breast cancer cells. Clin Exp
Metastasis.18, 139-46.
Nori, A., Kopecek, J., 2005. Intracellular targeting of polymer-bound drugs for cancer
chemotherapy. Adv. Drug. Deliv. Rev. 57, 609-636.
Nukolova, N.V., Oberoi, H.S., Cohen, S.M., Kabanov, A.V., Bronich, T.K., 2011.
Folate-decorated nanogels for targeted therapy of ovarian cancer. Biomaterials. 32,
Radheshyam, T., Kamla, P., 2011. Nanostructured lipid carrier versus solid lipid
nanoparticles of simvastain: Comparative analysis of characteristics,
pharmacokinetics and tissues uptake. Int J Pharm. 415, 232-243.
Rainer, M.H., 2007. Lipid nanoparticles: recent advances. Adv Drug Deliv Rev. 59,
Rainer, M.H., Cornelia, M.K., 2004. Challenges and solutions for the delivery of
biotech drugs-a review of drug nanocrystal technology and lipid nanoparticles. J of
Biotechnology. 113, 151-170.
Rosta L., 2002. Cold neutron research facility at the Budapest Neutron Centre. Appl
Phys. A. 74, S292-S294.
Schmidt, P. W., 1995. Some Fundamental Concepts and Techniques Useful in
Small-Angle Scattering Studies of Disordered Solids. In Modern Aspects of
Small-Angle Scattering; Brumberger, H., Ed.; Kluwer Academic Publishers: The
Netherlands, pp 1-56.
Seftor, R.E., Seftor, E.A. De, Larco, J.E., Kleiner, D.E., Leferson, J.,
Stetler-Stevenson, W.G., McNamara, T.F., Golub, L.M., Hendrix, M.J., 1998.
Chemically modified tetracyclines inhibit human melanoma cell invasion and
metastasis. Clin Exp Metastasis. 16, 217-25.
Selzer, M.G., Zhu, B., Block, N.L., Lokeshwar, B.L., 1999. CMT-3, a chemically
modified tetracycline, inhibits bony metastases and delays the development of
paraplegia in a rat model of prostate cancer. Ann N Y Acad Sci. 878, 678–682.
Soheila, K., Ebrahim, V.F., Mohsen, N., Fatemeh, A., 2010. Preparation and
characterization of ketoprofen-loaded solid lipid nanoparticles made from beeswax
and carnauba wax. Nanomedicine: Nanotechnology, Biology, and Medicine. 6,
Seydel, U., Brandenburg, K., Koch, M.H.J., Rietschel, E.T., 1989. Supramolecular
structure of lipopolysaccgaride and free lipid Aunder physiological conditions as
determined by synchrotron small-angle X-ray diffraction. Eur. J. Biochem. 186,
Syed S, Takimoto C, Hidalgo M, Rizzo J, Kuhn JG, Hammond LA, Schwartz G,
Tolcher A, Patnaik A, Eckhardt SG, Rowinsky EK. A phase I and pharmacokinetic
study of Col-3 (Metastat), an oral tetracycline derivative with potentmatrix
metalloproteinase and antitumor properties. Clin Cancer Res. 2004;
Sylvia, A.W., Rainer, M.H., 2004. Solid lipid mamoparticles for parenteral drug
delivery. Adv Drug Deliv Rev. 56, 1257-1272.
Torchilin, V.P., 2006. Recent approaches to intracellular delivery of drugs and DNA
and organelle targeting. Annu. Rev. Biomed. Eng. 8, 343-375.
Veerawat, T., Prapaporn, B., Eliana, B.S., Rainer, M.H., Varaporn, B.J., 2008.
Influence of oil content on physicochemical properties and skin distribution of Nile
red-loaded NLC. J Control Release. 128, 134-141.
Venkateraman, S., Hedrick, J.L., Ong, Z.Y., Yang, C., Ee, P.L.R., Hammond, P.T.,
Yang, Y.Y., 2011. The effects of polymeric nanostructure shape on drug delivery.
Adv Drug Deliv Rev. 63, 1228-1246.
Wang, J.J., Liu, K.S., Sung, K.C., Tsai, C.Y., Fang, J.Y., 2009. Lipid nanoparticles
with different oil/fatty eater ratios as carrier of buprenorphine and its prodrugs for
injection. Eur. J. Pharm. Sci. 38, 138-146.
Xu, Z.H., Chen, L.L., Gu, W.W., Gao,Y., Lin, L.P., Zhang, Z.W., Xi, Y., Li, Y.P., 2009.
The performance of docrtaxel-loaded solid lipid nanoparticles targeted to
hepatocellular carcinoma. Biomaterial. 30, 226-232.
Yoo, J.W., Doshi, N., Mitragotri, S., 2011. Adaptive micro and nanoparticles:
Temporal control over carrier properties to facilitate drug delivery. Adv Drug Deliv
Rev. 63, 1247-1256.
Zhigaltsev, I.V., Winters, G., Srinivasulu, M., Crawford, J., Wong, W., Amankwa, L.,
2010. Development of a weak-base docetaxel derivative that can be loaded into
lipid nanoparticles. J Control Release. 144, 332-340.
Figure Legends
Figure 1. The structures of doxycycline (upper) and CMT-3 (lower).
Fig 2. The effects of lipids and CMT-3 concentration on entrapment efficiency and
drug loading. *The labels of samples are the same as in Table. 3. Values are mean ±
Fig 3. AFM images of blank-NLC (A), and CMT/NLC (B).
Fig 4. The SEM photographs of blank-NLC (A) and CMT loaded NLC (B).
Fig 5. SANS spectra of NLC before and after loaded with CMT-3 in PBS (A); P(r)
function obtained from the corresponding scattering curves in A.
Fig 6. SAXS curve for film freeze-dried blank-NLC and CMT/NLC at 25 °C.
Fig 7. X-ray diffraction analysis of CMT-3 formulations: X-ray powder
diffractograms of CMT-3 (A), freeze-dried unloaded blank-NLC (B) and freeze-dried
Fig 8. The in-vitro release study. Cumulative release of CMT-3 from CMT/NLC in
different release mediums (A, Values are mean ± SD (n=3)), and average release rate
of CMT-3 from CMT/NLC in pH release medium (B).
Fig 9. In vitro cytotoxicity of CMT-3 and CMT/NLC against HeLa cells for 24 h (A)
or 48 h (B). Cell viability is expressed as the percentage of untreated controls. Data
are given as mean±SD (n=6). *p < 0.05 compared with CMT-3.
Fig 10. HeLa cells were treated with different medium for 6 h: A. control, without any
treatment; B. 20 μM CMT-3; C. blank-NLC (same volume with CMT/NLC); D.
CMT/NLC (CMT concentration is 20 μM). Cells were captured by digital camera
Fig 11. R1(A): control, without RB-NLC treatment; R1(B): HeLa cells were treated
with RB-NLC for 2 h; R2, 3: Red fluorescence from Rhodamine B, where the Hela
cells incubated with RB/NLC at the time of 0, 3, 6, 9,12, 15 minutes, respectively.
All images were captured by Cell'R Live Cell Station (×60).
Table legends
Table 1. Effects of lipid ingredients on mean particle size of blank-NLC.
Table 2. Effects of the concentration of surfactants on mean particle size and stability.
Table 3. The effects of lipids and CMT-3 content on mean particle size. Values are
mean ± SD (n=3)
Source: http://www.coastdat.eu/imperia/md/content/hzg/zentrale_einrichtungen/bibliothek/journals/2013/yang_30730.pdf
Control of Medical Journal Content: Suppressing the MBT Contamination Warning (Chapter 8, The Nurses are Innocent – The Digoxin Poisoning Fallacy, Dundurn Press, 2011) Key words: adverse reactions, Apotex, Avandia, benoxaprofen, deferiprone, drug companies, editorial interference, injection contamination, medical ethics, medical journals, MBT (mercapto-benzothiazole), Olivieri
Evaluations that make a Evaluations that make a difference-EN-20Sep15.indd 1 21/09/2015 13:14:17 Evaluations that make a difference-EN-20Sep15.indd 2 21/09/2015 13:14:18 extent possible, from the perspective The story of this project of the users and beneficiaries who have The issue of evaluation use is gaining been involved in the profiled evaluations