Eneuro.org
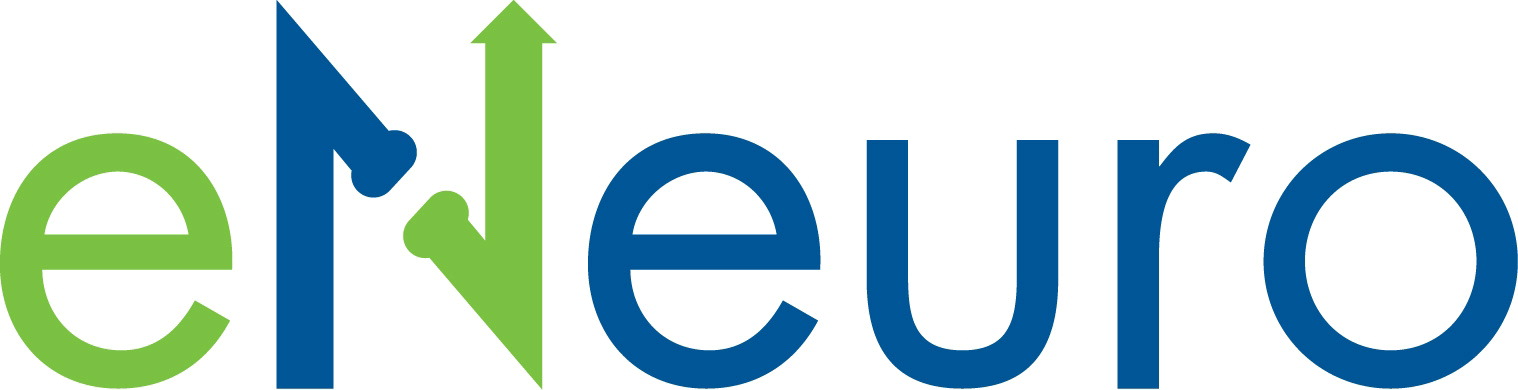

This Accepted Manuscript has not been copyedited and formatted. The final version may differ from this version.
Research Article: New Research Sensory and Motor Systems
Effects of fluoxetine and visual experience on glutamatergic and
GABAergic synaptic proteins in adult rat visual cortex
Effects of fluoxetine in adult rat V1
Simon Beshara , Brett R Beston
, Joshua GA Pinto
and Kathryn M Murphy
1McMaster Integrative Neuroscience Discovery and Study (MiNDS) Program, McMaster University2Department of Psychology, Neuroscience & Behavior, McMaster University3Department of Psychology, University of Toronto4Health Care Investment Banking, Credit Suisse AG
Received: 14 October 2015
Revised: 4 December 2015
Accepted: 8 December 2015
Published: 24 December 2015
Author Contributions: SB Analyzed data, wrote the paper; BB designed research, performed research,
analyzed data; JP designed research, performed research, analyzed data; KM designed research, analyzed
data, wrote the paper.
Funding: Gouvernement du Canada Natural Sciences and Engineering Research Council of Canada (Conseil
de Recherches en Sciences Naturelles et en Génie du Canada): 501100000038; RGPIN-2015-06215.
Conflict of Interest: Authors report no conflict of interest
NSERC Grant RGPIN-2015-06215 awarded to KM.
Corresponding Author: Kathryn M Murphy, McMaster Integrative Neuroscience Discovery and Study(MiNDS) Program, McMaster University, 1280 Main Street West, Hamilton, ON L8S 4K1, Canada. e-mail:[email protected]
Cite as: eNeuro 2015; 10.1523/ENEURO.0126-15.2015
Alerts: Sign up at eneuro.org/alerts to receive customized email alerts when the fully formatted version of this
article is published.
Accepted manuscripts are peer-reviewed but have not been through the copyediting, formatting, or proofreadingprocess.
This is an open-access article distributed under the terms of the Creative Commons Attribution 4.0 International(http://creativecommons.org/licenses/by/4.0), which permits unrestricted use, distribution and reproduction in anymedium provided that the original work is properly attributed.
Copyright 2015 Society for Neuroscience

Effects of fluoxetine and visual experience on glutamatergic and GABAergic
synaptic proteins in adult rat visual cortex
This is a confidential document and must not be discussed with others, forwarded in any
form, or posted on websites without the express written consent of eNeuro.

1. Manuscript Title: Effects of fluoxetine and visual experience on glutamatergic and
GABAergic synaptic proteins in adult rat visual cortex
2. Abbreviated Title: Effects of fluoxetine in adult rat V1
3. Authors: Simon Beshara1, Brett R Beston1,2,a, Joshua GA Pinto1,b, Kathryn M
1, McMaster Integrative Neuroscience Discovery and Study (MiNDS) Program,
McMaster University
2, Department of Psychology, Neuroscience & Behavior, McMaster University
a, Department of Psychology, University of Toronto
b, Health Care Investment Banking, Credit Suisse AG
4. Author Contributions:
SB Analyzed data, wrote the paper; BB designed research, performed research,
analyzed data; JP designed research, performed research, analyzed data; KM designed
research, analyzed data, wrote the paper.
5. Corresponding Author:
Kathryn M Murphy, McMaster Integrative Neuroscience Discovery and Study (MiNDS)
Program, McMaster University, 1280 Main Street West, Hamilton, ON L8S 4K1, Canada
e-mail: [email protected]
6. Number of Figures: 5
7. Number of Tables: 1
8. Number of multimedia: 0
9. Number of Words for Abstract: 205
10. Number of words for Significance Statement: 121
11. Number of words for Introduction: 574
12. Number of words for Discussion: 1948
13. Acknowledgements: None
14. Conflict of interest: Authors report no conflict of interest
15. Funding Sources: NSERC Grant RGPIN-2015-06215 awarded to KM

Fluoxetine has emerged as a novel treatment for persistent amblyopia because in adult
animals it reinstates critical period-like ocular dominance plasticity and promotes
recovery of visual acuity. Translation of these results from animal models to the clinic,
however, has been challenging because of the lack of understanding of how this SSRI
affects glutamatergic and GABAergic synaptic mechanisms that are essential for
experience-dependent plasticity. An appealing hypothesis is that fluoxetine recreates a
CP-like state by shifting synaptic mechanisms to be more juvenile. To test this we
studied the effect of fluoxetine treatment in adult rats, alone or in combination with
visual deprivation (monocular deprivation, MD), on a set of highly conserved pre- and
post-synaptic proteins (Synapsin, Synaptophysin, VGLUT1, VGAT, PSD-95, Gephyrin,
GluN1, GluA2, GluN2B, GluN2A, GABAAα1, GABAAα3). We did not find evidence that
fluoxetine shifted the protein amounts or balances to a CP-like state. Instead, it drove
the balances in favor of the more mature subunits (GluN2A, GABAAα1). In addition,
when fluoxetine was paired with MD it created a neuroprotective-like environment by
normalizing the glutamatergic gain found in adult MDs. Together our results suggest
that fluoxetine treatment creates a novel synaptic environment dominated by GluN2A-
and GABAAα1-dependent plasticity.

Patching therapy is the most common treatment for children with amblyopia. For
many, the acuity recovered during patching is lost when the treatment stops leaving the
child with persistent amblyopia. Fluoxetine has emerged as an interesting treatment
option because it reinstates critical period-like ocular dominance plasticity and
promotes acuity recovery in adult animals. It remains unclear, however, how this
selective serotonin reuptake inhibitor affects visual cortex plasticity, which relies
heavily on glutamatergic and GABAergic synapses. Here we report the effects of
fluoxetine and visual manipulation on the visual cortex of adult rats. Surprisingly we
found that fluoxetine did not reinstate a critical period-like state, but rather created a
novel synaptic environment that favors mature NMDA and GABAA receptor subunits.

Amblyopia is commonly treated with patching but in some cases the recovered
acuity is lost when patching stops, leaving the child with persistent amblyopia (Birch,
2013). A variety of therapeutics have been proposed to treat persistent amblyopia in
adolescents or young adults. Fluoxetine has emerged as a treatment option because it
reinstates critical period-like (CP) ocular dominance plasticity and promotes acuity
recovery in adult rats (Vetencourt et al., 2008). It is unclear, however, what effects this
selective serotonin reuptake inhibitor (SSRI) has on visual cortex (V1) plasticity that
relies heavily on maturation of glutamatergic and GABAergic synapses (Levelt and
Hübener, 2012). An appealing idea is that fluoxetine shifts the synaptic environment in
V1 to a CP-like state that supports heightened experience-dependent plasticity.
During the CP, experience-dependent plasticity is driven by visually evoked
responses that depend upon maturation of pre- and post-synaptic mechanisms.
Development of presynaptic vesicle cycling proteins (e.g. Synapsin, Synaptophysin) and
transporters (e.g. VGLUT, VGAT) are necessary for reliable neurotransmitter release
(Hopf et al., 2002; Conti et al., 2004; Wojcik et al., 2004; 2006) that drives strong visually
evoked activity. Also, shifts in the excitation:inhibition (E/I) balance set up the
physiological environment needed for heightened plasticity, triggering the CP (Hensch,
2005; Hensch and Fagiolini, 2005). That E/I balance is mediate by postsynaptic
scaffolding proteins PSD-95 and Gephyrin that regulate the number of excitatory and
inhibitory synapses (Prange et al., 2004; Lardi-Studler et al., 2007; Keith and El-

Husseini, 2008). Furthermore, the start of the CP in rat and human V1 coincides with a
rapid switch from much more Gephyrin to an equal balance with PSD-95 (Pinto et al.,
A host of glutamatergic and GABAergic receptor mechanisms affect the threshold
for CP plasticity. These include addition of AMPA receptors (AMPAR) that end the
period of NMDA receptor (NMDAR)-dominated silent synapses (Huang et al., 2015)
and add the fast component to excitatory postsynaptic currents (EPSCs) (Kleppe and
Robinson, 1999). Furthermore, the addition of GluN2A containing NMDARs (Flint et
al., 1997; Stocca and Vicini, 1998) speeds up receptor kinetics (Cull-Candy et al., 2001)
and affects signalling pathways such as GluN2B activation of Ras/ERK or alpha
calcium-calmodulin kinase II and mTOR pathways (Kim et al., 2005; Wang et al., 2011).
The shift to GluN2A also affects functional maturation by mediating sharpening of
orientation selectivity (Fagiolini et al., 2003). Finally, activation of GABAA receptors
(GABAAR) triggers the start of the CP (Hensch, 2005) and GABAAα1 subunits in
particular regulate patterns of activity needed for development of ocular dominance
(Fagiolini et al., 2004).
Despite our understanding of the influence of fluoxetine treatment on adult
plasticity, there is little evidence to identify 'how' fluoxetine affects the expression
profile of synaptic mechanism that are critical in the initiation of CP plasticity. To
address this, we treated animals with fluoxetine and quantified a set of glutamatergic
and GABAergic synaptic proteins to assess if they changed to a CP-like state. We then
determined the effects of monocular deprivation (MD) alone, or in combination with
fluoxetine on these synaptic proteins. Surprisingly, fluoxetine alone shifted both
NMDAR and GABAAR subunits to a more mature composition. Furthermore, when
fluoxetine was combined with MD, the treatment normalized the increase in
glutamatergic proteins found in adult MD rats. These results show that fluoxetine
treatment does not recreate a CP-like synaptic environment but instead shifts plasticity
mechanisms to a new state.
Materials and Methods
Animals and surgical procedures
We studied changes in expression of 12 synaptic proteins in V1 of young adult male
Long Evans rats (P98). Rats were individually housed in plexiglas cages with food and
water ad libitum, and a 12:12 light/dark cycle. Animals were randomly assigned to 1 of
4 groups: normally reared to P98 (n=6), animals given 4 weeks of fluoxetine (from P70-
P98) (0.2mg/ml of drinking water) (n=8), animals monocularly deprived (MDed) (P91-
P98) (n=6), or animals that received both fluoxetine (P70-P98) and MD (P91-P98)(n=8).
Eyelids were closed by trimming the lid margins and suturing them together with 5-0
vicryl using aseptic surgical techniques. The surgery was done using gaseous
anesthetic [isoflurane (1.5–5%) in oxygen] for induction and maintenance of anesthesia.
Eyelids were inspected daily for openings. All experimental procedures were approved
by the [Authors' University] Animal Research Ethics Board.
Tissue collection
Animals were euthanized (sodium pentobarbital, 150 mg/kg), and transcardially
perfused with cold 0.1 M phosphate buffered saline (PBS) (4°C; 4-5 ml/min) until
circulating fluid was clear. The brain was quickly removed from the skull and
immersed in cold PBS. Bilateral samples of V1 including monocular and binocular
regions, quickly frozen on dry ice, and stored at -80°C.
Sample preparation
To study high-abundance vesicle cycling proteins and receptor scaffolding proteins
(Synapsin, Synaptophysin, PSD-95, Gephyrin) we prepared homogenate samples. The
frozen tissue was added to cold homogenization buffer (1 ml buffer:50 mg tissue – 0.5
mM DTT, 1mM EDTA, 2 mM EGTA, 10 mM HEPES, 10 mg/L leupeptin, 100 nM
microcystin, 0.1 mM PMSF, 50 mg/L soybean trypsin inhibitor) and homogenized in a
glass-glass Dounce homogenizer (Kontes, Vineland, NJ, USA). The sample was then
combined with 10% sodium-dodecylsulfate (SDS). To study lower abundance receptor
subunits (GluA2, GluN1, GluN2A, GluN2B, GABAAα1, GABAAα3) and transporters
(VGLUT1, VGAT) we enriched the samples following a synaptoneurosomes protocol
(Hollingsworth et al., 1985; Quinlan et al., 1999; Murphy et al., 2014). Following the
homogenization step each sample was passed through a 5μm pore hydrophilic mesh
filter (Millipore, Bedford, MA), then centrifuged at 1,000 g for 10 min. Both the synaptic
pellet and the whole homogenate samples were resuspended in boiling 1% SDS. Protein
concentrations for each sample was determined using the bicinchoninic acid (BCA)
assay guidelines (Pierce, Rockford, IL, USA) and final concentrations were adjusted to
1mg/ml using Laemmli sample buffer. A control sample was made by combining a
small amount of each of the 28 samples.
Samples (25 μg) were resolved on 4-20% SDS–polyacrylamide gel electrophoresis
(SDS–PAGE) gels (Precise Protein Gels, Pierce Biotechnology Inc., Rockford, IL, USA)
and transferred to polyvinylidene difluoride (PVDF-FL) membranes (Millipore,
Billerica, MA, USA). Blots were incubated with blocking buffer (Odyssey Blocking
Buffer 1:1 with PBS) for 1 hour (LI-COR Biosciences; Lincoln, NE, USA), then with
primary antibody overnight at 4°C using the following concentrations: GAPDH, 1:4000
(Imgenex, San Diego, CA); Synapsin 1, 1:8000 (Invitrogen, Carlsbad, CA);
Synaptophysin, 1:2000 (Sigma-Aldrich, St. Louis, MO); PSD-95, 1:32000 (Millipore,
Billerica, MA); Gephyrin, 1:2000 (Millipore, Billerica, MA); VGLUT1 , 1:2000 (Synaptic
Systems, Goettingen, Germany); VGAT, 1:2000 (Synaptic Systems, Goettingen,
Germany); GluA2, 1:2000 (Invitrogen, Carlsbad, CA); GluN1, 1:8000 (Chemicon
International, Temecula, CA); GluN2B, 1:2000 (Chemicon International, Temecula, CA);
GluN2A, 1:2000 (PhosphoSolutions, Aurora, CO); GABAAα1 1:500 (Santa Cruz
Biotechnology, Santa Cruz, CA, USA); GABAAα3 1:2000 (Chemicon International,
Temecula, CA, USA). The blots were washed with PBS containing 0.05% Tween (Sigma,
St. Louis, MO, USA) (PBS-T; 3 × 10 min), incubated for 1 hour at room temperature with
the appropriate IRDye labeled secondary antibody, (Anti-Mouse, 1:8000, Anti-Rabbit,
1:10,000; LI-COR Biosciences; Lincoln, NE, USA), and washed in PBS-T (3 × 10 min).
The blots were visualized using an Odyssey scanner (LI-COR Biosciences; Lincoln, NE,
USA). The combination of IRDye secondary antibodies and Odyssey scanner provides a
wide linear dynamic range so that both strong and weak bands could be accurately
quantified on the same blot. Blots were stripped and re-probed with additional
antibodies (Blot Restore Membrane Rejuvenation kit, Millipore, Billerica, MA, USA).
Analyses
To analyze the bands, we scanned the blots (Odyssey infrared scanner) and
quantified the bands using densitometry (LI-COR Odyssey Software version 3.0; LI-
COR Biosciences; Lincoln, NE, USA). Density profiles were determined by performing a
subtraction of the background, integrating the pixel intensity across the area of the
band, and dividing the intensity by the width of the band to control for variations in
lane width. Protein loading was checked using GAPDH as a control for sample
concentration and volume loaded in each well. Each band was normalized to the
average for the set of blots run at the same time and the control sample on the
individual blot.
To quantify the relationship between functional pairs of proteins we calculated
contrast indices that are commonly used in signal processing to determine the quality of
the signal. AMPAR-NMDAR Index -- (GluA2-GluN1)/(GluA2+GluN1). NMDAR Index
-- (GluN2A-GluN2B)/(GluN2B+GluN2A). GABAAR Index -- (GABAA α1-GABAA
α3)/(GABAA α1+GABAA α3). Presynaptic E/I Index -- (VGLUT1-
VGAT)/(VGLUT1+VGAT). Postsynaptic E/I Index -- (PSD-95-Gephyrin)/(PSD-
To compare levels of protein expression among the groups we made histograms
showing the mean and standard error of the mean for each group. All results were
plotted normalized to the normal young adult group. To make statistical comparisons
between groups we used bootstrapping, a modern resampling statistical method that
provides robust estimates of standard error and confidence intervals, that is especially
useful for animal studies such as ours constrained to smaller sample sizes. We used R to
simulate a normally distributed data set with 1,00,000 points and the same mean and
standard deviation as the group being compared. To determine differences between
groups we compared the simulation data set with average protein expression with each
of the other groups. We ran a Monte Carlo simulation which randomly samples from
the simulation data set N time, where N was the number of animals in each of the other
groups (N=6 or 8). This simulation was repeated 10,000 times to create the normal
distribution expected for the N sample sizes. We calculated confidence intervals for the
simulated distribution and compared those with the observed means for the other
groups. Groups were identified as significantly different (e.g. p < 0.05) when the
observed average expression was either greater or less than 95% of the simulated
distribution and thus outside its confidence interval.
Image Manipulation
Bands are representative samples taken from different parts of the same gel or
different gels. Horizontal and vertical transformations were uniformly applied to size
bands appropriately for each figure. A linear adjustment layer was applied uniformly to
all bands of each protein, preserving the relative intensities between groups.
We verified that GAPDH was an appropriate loading control by comparing
expression of it among the 4 groups. We found no significant differences from normals
demonstrating that GAPDH is an appropriate loading control. We began by examining
expression of Synapsin, Snaptophysin, PSD-95 and Gephyrin in V1 ipsilateral to the
deprived eye. MD effects are much weaker in the ipsilateral hemisphere (Sawtell et al.,
2003) and we did not find any significant differences among the groups for those
synaptic proteins (Fig. 1). Thus, all of the following analyses are for V1 contralateral to
the deprived eye.
Presynaptic changes
We analyzed how fluoxetine changed the presynaptic environment by quantifying a
set of proteins involved in cycling, transport and loading of glutamatergic and
GABAergic vesicles. We compared expression of Synapsin, Synaptophysin, VGLUT1
and VGAT in V1 of normally reared adults rats, rats given 1 month of fluoxetine, 1
week of MD, or the combination of fluoxetine and MD. We found no differences
among the groups for Synapsin (n.s.) (Fig. 2A) or the GABAergic transporter VGAT
(n.s.) (Fig. 2D) and only a modest loss of Synaptophysin for the MDed animals (-13%
SEM 4.1%, p < 0.05) (Fig. 2B). The glutamate transporter VGLUT1 , however, had more
changes. MDed animals had an increase in VGLUT1 (+25% SEM 8.4%, p < 0.001) while
both groups of fluoxetine treated animals had less VGLUT1 than normal (fluoxetine
alone -29% SEM 3.0%, p < 0.0001; fluoxetine+MD -13% SEM 4.9%, p < 0.05) (Fig. 2C).
Postsynaptic changes
Next we examined how fluoxetine changed the expression of a set of postsynaptic
scaffolding proteins and receptor subunits for glutamatergic and GABAergic receptors.
Changes among the groups were very similar for PSD-95 and Gephyrin. Fluoxetine
alone did not change the level of expression relative to normal animals, but MD caused
loss of expression (PSD-95 -37% SEM 5.6%, p 0.06; Gephyrin -45% SEM 4.0%, p < 0.01)
and fluoxetine combined with MD increased expression (PSD-95 +46% SEM 15%, p <
0.05; Gephyrin +34% SEM 11%, p < 0.05) (Fig. 3A-B).
The pattern of changes for the receptor subunits was almost opposite to the
scaffolding proteins. For the glutamatergic receptor subunits (GluN1, GluA2, GluN2B,
GluN2A) fluoxetine alone caused losses for GluN1 and GluN2B (GluN1 -15% SEM
4.8%, p < 0.01; GluN2B -28% SEM 4.5 %, p < 0.01) and when combined with MD caused
a loss of GluA2, as well as losses for GluN1 and GluN2B (GluA2 -15% SEM 4.2%, p <
0.0001; GluN1 -18% SEM 4.5%, p < 0.0001; GluN2B -21% SEM 4 %, p < 0.05) (Fig. 3C-F).
But MD alone caused either an increase (GluN1 +25% SEM 8.8%, p < 0.0001; GluA2
+18% SEM 5.9%, p < 0.05) or no significant change from normal (GluN2B, GluN2A,
n.s.). Thus, MD alone caused gains for these glutamatergic subunits that were reduced
when MD was combined with fluoxetine. MD also increased GABAAα3 (+18% SEM
6.6%, p < 0.001) (Fig. 3G) but did not change GABAAα1 (Fig. 3H). In contrast, GABAAα1
was increased in both fluoxetine treated groups (fluoxetine alone +24% SEM 11%, p <
0.001; fluoxetine+MD +24% SEM 20%, p < 0.001) (Fig. 3H).
Receptor subunit balances
During development there are a series of maturational shifts in expression of
glutamatergic and GABAergic receptor subunits. One of the shifts is the change from
NMDAR-dominated silent synapses to AMPAR activated synapses. We studied if
fluoxetine created a CP-like state by shifting the GluA2:GluN1 balance in favour of
GluN1 but found no changes from the normal adult balance (n.s.) (Fig. 4A). Different
results were found when the GluN2A:GluN2B and GABAAα1:GABAAα3 balances were
examined. During normal development there is an increase in GluN2A, shifting the
balance from much more GluN2B to slightly in favour of GluN2B in young adult rats
(Fig. 4B). But all of the experimental groups changed beyond that level towards even
more GluN2A (p < 0.05). There were differences, however, in what drove the changes
in the GluN2A:GluN2B balance with the fluoxetine groups shift being caused by less
GluN2B while the MD shift was caused by more GluN2A. The GABAAα1:GABAAα3
balance revealed another dissociation among the experimental groups (Fig. 4C). Here
the MD shift was caused by a 20% increase in GABAAα3 (p < 0.05), while the shift for
the fluoxetine groups was caused by a 20% increase in GABAAα1 (fluoxetine alone, p <
0.01; fluoxetine+MD, p < 0.05) (Fig. 4C). This series of subunit balances unpacks subtle
effect of fluoxetine treatment showing that it does not cause a shift to a CP-like state,
instead it maintains subunit balances that are like normal adults (GluA2:GluN1) or
shifted to more of the mature subunits (GluN2A, GABAAα1).
E/I balances
The final analyses examined pre- and post-synaptic proteins that regulate the E/I
balance. First, we calculated a presynaptic E/I balance using the vesicular transporters
VGLUT1 and VGAT. MD caused a large shift towards VGLUT1 (p < 0.05) (Fig. 5A) but
when combined with fluoxetine there was no change in the presynaptic E/I balance.
The same pattern was seen on the postsynaptic side, here MD also caused a large shift
towards the excitatory side (more PSD-95) (p < 0.05) (Fig. 5B) but when MD was paired
with fluoxetine there was no change from the normal adult E/I balance.
In this study, we quantified the effect of fluoxetine treatment on 12 glutamatergic
and GABAergic markers linked with visual experience-dependent plasticity in V1.
Fluoxetine caused a pattern of change in those markers that provides new insights into
how this drug affects plasticity in adult V1. We compared normal adult rats with ones
treated with either fluoxetine alone, MD, or fluoxetine paired with MD. The main
findings are that fluoxetine treatment in adult rats does not shift these markers to a
younger pattern but instead rebalances MD driven glutamatergic gain and promotes a
novel synaptic environment.
In this study, we used Western blotting to quantify the effects of fluoxetine
treatment on a collection of synaptic proteins in adult V1. A strength of this approach is
that a large number of synaptic proteins were analyzed. Western blotting, however,
does not provide laminar or cell specific information that is needed to identify the
neural circuits in V1 affected by fluoxetine. Future neuroanatomical studies are needed
to address that question and those studies may be guided by the current results.
Fluoxetine does not recreate a younger synaptic environment
An appealing hypothesis about drug treatments, such as fluoxetine, is that they re-
instate ocular dominance plasticity in adult V1 by changing the synaptic environment to
a CP-like state. During the CP there are increases in amount of proteins and shifts in
balances between functional pairs of synaptic proteins. Our results do not support the
idea that fluoxetine in adult rats dials back synaptic age. For example, we found that
fluoxetine combined with MD caused greater expression of PSD-95 and Gephyrin.
These levels were higher than found during the CP (Pinto et al., 2015) and are consistent
with a spike in PSD-95 that ends the CP (Huang et al., 2015). Furthermore, fluoxetine
alone did not reduce expression of either scaffolding protein and only MD caused a loss
of PSD-95 and Gephyrin. The modest losses for VGLUT1 and receptor subunits caused
by fluoxetine suggest a shift to a more immature stage, but the balances among the
subunits do not support that conclusion. If a younger synaptic environment was
recreated then it should favour NMDA over AMPA receptors (Wu et al., 1996), GluN2B
over GluN2A (Carmignoto and Vicini, 1992; Flint et al., 1997; Stocca and Vicini, 1998)
and GABAAα3 over GABAAα1 (Laurie et al., 1992). Instead, the NMDAR to AMPAR
ratios were balanced for both fluoxetine groups (GluN1 GluA2), while subunit
balances jumped past age matched adults towards even more of the mature subunits
(GluN2A, GABAAα1). Finally, E/I balances for pre- and post-synaptic markers were
similar to the normal adults in both fluoxetine groups. Together these findings
illustrate that fluoxetine treatment did not simply recreate a CP-like synaptic
environment in V1.
It is important to note that we examined synaptic proteins after 1 month of
fluoxetine treatment and 1wk of MD. We know from previous studies (Williams et al.,
2015) that there are dynamic changes in synaptic proteins during a period of MD and it
seems reasonable to propose that fluoxetine treatment may cause similarly dynamic
changes. Thus the findings here provide a snapshot of long-term effects of fluoxetine
treatment. It will be important for future studies to probe other time points to
understand the full landscape of synaptic changes and how transient changes caused by
fluoxetine (Vetencourt et al., 2011) impact long-term plasticity in V1.
Fluoxetine triggers a novel synaptic environment in adult V1
The original study showing that fluoxetine reinstates ocular dominance plasticity
also found improvement of visual function, reduced intracortical inhibition, and
increased BDNF expression (Vetencourt et al., 2008). All of those changes occurred
without significantly altering neuronal responsiveness or orientation selectivity in V1.
Here we found normal pre- and post-synaptic E/I balances, and adult levels of
GABAAα1 that could support normal responsiveness and orientation selectivity. A
previous study of gene expression found reduced VGAT after fluoxetine treatment but
no changes in other genes associated with E/I mechanisms (Tiraboschi et al., 2013). We,
however, did not find that fluoxetine caused a loss of VGAT protein expression. Some
forms of GABAergic plasticity involve changes in VGAT protein expression associated
with the amount of neurotransmitter in vesicles (Hartman et al., 2006), and the lack of
change in VGAT makes it unlikely that fluoxetine altered this type of plasticity.
A recent proteomic analysis found that fluoxetine caused alterations in cytoskeleton
organization, endocytosis, molecular transport, intracellular signaling, redox cellular
state, metabolism and protein degradation (Ruiz-Perera et al., 2015). Those changes
included proteins that regulate AMPAR and GABAAR, and may affect the E/I balance.
Nonetheless, our quantification of synaptic proteins, along with the gene and proteomic
studies, show that fluoxetine affects mechanisms that regulate experience-dependent
The GluN2A:GluN2B and GABAAα1:GABAAα3 balances were both affected by
fluoxetine and importantly the GABAA balance differentiated fluoxetine treatments
from the effects of MD. The changes in these functional pairs of glutamatergic and
GABAergic receptor subunits suggest that fluoxetine creates a novel synaptic
environment in adult V1. An environment that is dominated by GluN2A and
GABAAα1 but also has balanced levels of pre- and post-synaptic E/I markers. Both
GluN2A and GABAAα1 subunits are described as mature components because they
gradually increase during development and affect plasticity. For example, the
developmental shift from GluN2B to more GluN2A speeds up receptor kinetics (Cull-
Candy et al., 2001), changes cellular signalling (Kim et al., 2005; Wang et al., 2011),
relieves GluN2B negative regulation of AMPARs (Hall and Ghosh, 2008), and controls
metaplasticity in V1 (Philpot et al., 2007). GABAAα1 is necessary for normal
development of orientation tuning (Fagiolini et al., 2004) and gamma rhythms (Cardin
et al., 2009; Sohal et al., 2009). The prevalence of GABAAα1 positive synapses on
pyramidal cell bodies makes them important components in GABAergic regulation of
experience-dependent plasticity (Hensch, 2005; Griffen and Maffei, 2014). The different
roles of these subunits in experience-dependent plasticity suggests that fluoxetine
creates a unique synaptic environment in adult V1 that can support both GluN2A-
dependent metaplasticity and GABAergic regulation of ocular dominance plasticity.
How might fluoxetine trigger adult plasticity?
Reduced intracortical GABA and GABAergic transmission have been found after
fluoxetine treatment (Vetencourt et al., 2008; Baroncelli et al., 2011). In contrast, we
found a small increase in GABAAα1 expression and no loss of GABAAα3 or VGAT in
rats treated with fluoxetine. Previous studies have shown that fluoxetine positively
modulates GABAA receptors and one way is by increasing receptor sensitivity to small
amounts of GABA (Robinson, 2002). The α1 subunit is one of the subtypes that confers
that increased sensitivity and perhaps more GABAAα1 expression modulates
GABAergic drive when the amount of neurotransmitter is reduced by fluoxetine.
Interestingly, during the CP a brief exposure to vision after deprivation causes a rapid
rebound potentiation in mini inhibitory postsynaptic currents (mIPSCs) that is
correlated with an increase in GABAARs (Gao et al., 2014). Perhaps the increase in
GABAAα1 expression found here supports a similar potentiation of mIPSCs and since
GABAAα1 containing synapses form a key part of the neural circuitry involved in ocular
dominance plasticity (Hensch, 2005) fluoxetine may drive a compensatory mechanisms
where sensitized GABAARs enhance adult plasticity.
We also found that fluoxetine caused changes to glutamatergic receptor subunit
expression. Fluoxetine is known to inhibit NMDA receptors and may provide
neuroprotective effects by regulating glutamatergic involvement in excitotoxicity (Szasz
et al., 2007). We found that fluoxetine paired with MD ameliorated glutamatergic gain
driven by MD alone, suggesting that one of fluoxetine's effects in adult V1 may be
neuroprotective. Fluoxetine acts by inhibiting GluN2B containing NMDARs (Kiss et al.,
2012) and that may trigger increases in both BDNF and AMPARs. GluN2B-mediated
signalling inhibits AMPAR trafficking and the amount of GluA2 containing AMPARs
(Kim et al., 2005; Derkach et al., 2007; Hall et al., 2007; Hall and Ghosh, 2008) through
unique cellular processes that include Ras/ERK, αCamKII, and mTor pathways (Kim et
al., 2005; Wang et al., 2011). One way that fluoxetine could affect adult ocular
dominance plasticity is if the loss of GluN2B changes the length of GluN2B-mediated
Ras/ERK activation (Kim et al., 2005) thereby increasing insertion of AMPAR into
synapses and supporting long-term potentiation (LTP). ERK activation is necessary for
ocular dominance plasticity in developing V1 (Di Cristo et al., 2001) and fluoxetine in
adult animals may enhance ERK-dependent plasticity through the loss of GluN2B.
During the CP ocular dominance plasticity reflects the depression of deprived eye
responses but in adults MD leads to enhancement of open eye responses in V1 (Sawtell
et al., 2003). That adult plasticity is dependent on activation of NMDARs and may use
hebbian (LTP, long-term depression LTD, spike time dependent plasticity), homeostatic,
or metaplasticity (synaptic modification threshold) mechanisms (for review, see Hofer
et al., 2006). Visual experience driven changes to LTP and LTD during the CP depend
on GluN2A and previous studies have identified shifts in the GluN2A:GluN2B balance
as the mechanism underlying an adjustable synaptic modification threshold in V1
(Philpot et al., 2007). Perhaps the shift to balanced GluN2A:GluN2B expression after
fluoxetine treatment is an indication that metaplasticity plays a dominant role in
fluoxetine driven adult plasticity. Interestingly, in auditory cortex fluoxetine reduces
the potential for LTP (Dringenberg et al., 2014) raising the possibility that the effects of
fluoxetine might not be uniform across the cortex.
Fluoxetine could also trigger events similar to those promoted by other NMDAR
antagonists that cause a transient burst of glutamate, followed by BDNF release and
synapse formation (Duman and Aghajanian, 2014). BDNF plays a key role in
fluoxetine's reactivation of plasticity (Castrén and Rantamäki, 2010) suggesting that a
fluoxetine induced loss of GluN2B signalling may enhance BDNF and AMPAR
involvement in experience-dependent plasticity in adult V1. Thus, fluoxetine appears
to enhance glutamatergic and GABAergic mechanisms that support experience-
dependent plasticity in adult V1.
Implications for other therapies
A variety of other methods are being explored to promote adult recovery from
persistent amblyopia, such as dark rearing in animals (He et al., 2006; 2007; Montey and
Quinlan, 2011; Duffy and Mitchell, 2013), manipulation of the brakes on plasticity
including PirB (Bochner et al., 2014) and chondroitin sulphate proteoglycans (CSPGs)
(Pizzorusso et al., 2002; Morishita et al., 2010; Bukhari et al., 2015), environmental
enrichment (Sale et al., 2007), patterned visual stimulation (Montey et al., 2013), or
perceptual learning (Levi and Li, 2009; Baroncelli et al., 2011; Bonaccorsi et al., 2014;
Tsirlin et al., 2015). All of these appear to reactivate a certain degree of plasticity that
can support ocular dominance plasticity and even visual recovery. The cellular
mechanisms typically include LTP of cortical synapses, and although some molecular
changes have been identified (He et al., 2006), the full extent has yet to be explored. Do
these other techniques mimic the novel pattern of fluoxetine driven glutamatergic and
GABAergic changes or do they create different synaptic environments? These are
important questions to answer to determine if these adult manipulations activate one or
many different forms of experience-dependent plasticity in V1.
Future studies will need to determine the long-term consequences of fluoxetine-
induced changes in adult V1. It is not clear if stopping drug treatment will allow the
synaptic environment to shift back to a normal adult state. And if not what effects that
new synaptic environment may have on neural function in the long-term. Finally, it will
be important to determine how much of these effects are driven by the increase in
serotonin, as opposed to unique effects of fluoxetine. Each of these are important
questions to answer that well help to understand plasticity in adult V1 and translate
that knowledge into effective treatments for persistent amblyopia.
Baroncelli L, Maffei L, Sale A (2011) New perspectives in amblyopia therapy on adults:
a critical role for the excitatory/inhibitory balance. Front Cell Neurosci 5:25.
Birch EE (2013) Amblyopia and binocular vision. Prog Retin Eye Res 33:67–84.
Bochner DN, Sapp RW, Adelson JD, Zhang S, Lee H, Djurisic M, Syken J, Dan Y, Shatz
CJ (2014) Blocking PirB up-regulates spines and functional synapses to unlock
visual cortical plasticity and facilitate recovery from amblyopia. Sci Transl Med
Bonaccorsi J, Berardi N, Sale A (2014) Treatment of amblyopia in the adult: insights
from a new rodent model of visual perceptual learning. Front Neural Circuits
Bukhari N, Burman PN, Hussein A, Demars MP, Sadahiro M, Brady DM, Tsirka SE,
Russo SJ, Morishita H (2015) Unmasking Proteolytic Activity for Adult Visual
Cortex Plasticity by the Removal of Lynx1. J Neurosci 35:12693–12702.
Cardin JA, Carlén M, Meletis K, Knoblich U, Zhang F, Deisseroth K, Tsai L-H, Moore CI
(2009) Driving fast-spiking cells induces gamma rhythm and controls sensory
responses. Nature 459:663–667.
Carmignoto G, Vicini S (1992) Activity-dependent decrease in NMDA receptor
responses during development of the visual cortex. Science 258:1007–1011.
Castrén E, Rantamäki T (2010) The role of BDNF and its receptors in depression and
antidepressant drug action: Reactivation of developmental plasticity. Dev
Neurobiol 70:289–297.
Conti F, Minelli A, Melone M (2004) GABA transporters in the mammalian cerebral
cortex: localization, development and pathological implications. Brain Res Rev
Cull-Candy S, Brickley S, Farrant M (2001) NMDA receptor subunits: diversity,
development and disease. Curr opin neurobiol 11:327–335.
Derkach VA, Oh MC, Guire ES, Soderling TR (2007) Regulatory mechanisms of AMPA
receptors in synaptic plasticity. Nat Rev Neurosci 8:101–113.
Di Cristo G, Berardi N, Cancedda L, Pizzorusso T, Putignano E, Ratto GM, Maffei L
(2001) Requirement of ERK Activation for Visual Cortical Plasticity. Science
292:2337–2340.
Dringenberg HC, Branfield Day LR, Choi DH (2014) Chronic Fluoxetine Treatment
Suppresses Plasticity (Long-Term Potentiation) in the Mature Rodent Primary
Auditory Cortex In Vivo. Neural Plast 2014:571285.
Duffy KR, Mitchell DE (2013) Darkness Alters Maturation of Visual Cortex and
Promotes Fast Recovery from Monocular Deprivation. Current Biology 23:382–
Duman RS, Aghajanian GK (2014) Neurobiology of rapid acting antidepressants: role of
BDNF and GSK-3β. Neuropsychopharmacol 39:233–252.
Fagiolini M, Fritschy J-M, Low K, Mohler H, Rudolph U, Hensch TK (2004) Specific
GABAA Circuits for Visual Cortical Plasticity. Science 303:1681–1683.
Fagiolini M, Katagiri H, Miyamoto H, Mori H, Grant SGN, Mishina M, Hensch TK
(2003) Separable features of visual cortical plasticity revealed by N-methyl-d-
aspartate receptor 2A signaling. Proc Natl Acad Sci USA 100:2854–2859.
Flint AC, Maisch US, Weishaupt JH, Kriegstein AR, Monyer H (1997) NR2A Subunit
Expression Shortens NMDA Receptor Synaptic Currents in Developing
Neocortex. J Neurosci 17:2469–2476.
Gao M, Maynard KR, Chokshi V, Song L, Jacobs C, Wang H, Tran T, Martinowich K,
Lee H-K (2014) Rebound Potentiation of Inhibition in Juvenile Visual Cortex
Requires Vision-Induced BDNF Expression. J Neurosci 34:10770–10779.
Griffen TC, Maffei A (2014) GABAergic synapses: their plasticity and role in sensory
cortex. Front Cell Neurosci:1–22.
Hall BJ, Ghosh A (2008) Regulation of AMPA receptor recruitment at developing
synapses. Trends Neurosci 31:82–89.
Hall BJ, Ripley B, Ghosh A (2007) NR2B Signaling Regulates the Development of
Synaptic AMPA Receptor Current. J Neurosci 27:13446–13456.
Hartman KN, Pal SK, Burrone J, Murthy VN (2006) Activity-dependent regulation of
inhibitory synaptic transmission in hippocampal neurons. Nat Neurosci 9:642–
He H-Y, Hodos W, Quinlan EM (2006) Visual Deprivation Reactivates Rapid Ocular
Dominance Plasticity in Adult Visual Cortex. J Neurosci 26:2951–2955.
He H-Y, Ray B, Dennis K, Quinlan EM (2007) Experience-dependent recovery of vision
following chronic deprivation amblyopia. Nat Neurosci 10:1134–1136.
Hensch TK (2005) Critical period plasticity in local cortical circuits. Nat Rev Neurosci
Hensch TK, Fagiolini M (2005) Excitatory–inhibitory balance and critical period
plasticity in developing visual cortex. In: Development, Dynamics and
Pathiology of Neuronal Networks: from Molecules to Functional Circuits, pp
115–124 Progress in Brain Research. Elsevier.
Hofer SB, Mrsic-Flogel TD, Bonhoeffer T, Hübener M (2006) Lifelong learning: ocular
dominance plasticity in mouse visual cortex. Curr opin neurobiol 16:451–459.
Hollingsworth EB, McNeal ET, Burton JL, Williams RJ, Daly JW, Creveling CR (1985)
Biochemical characterization of a filtered synaptoneurosome preparation from
guinea pig cerebral cortex: cyclic adenosine 3":5-"monophosphate- generating
systems, receptors, and enzymes. J Neurosci 5:2240–2253.
Hopf W, Waters J, Mehta S, Smith SJ (2002) Stability and Plasticity of Developing
Synapses in Hippocampal Neuronal Cultures. J Neurosci 22:775–781.
Huang X, Stodieck SK, Goetze B, Cui L, Wong MH, Wenzel C, Hosang L, Dong Y,
Löwel S, Schlüter OM (2015) Progressive maturation of silent synapses governs
the duration of a critical period. Proc Natl Acad Sci USA 112:E3131–E3140.
Keith D, El-Husseini A (2008) Excitation Control: Balancing PSD-95 Function at the
Synapse. Front Mol Neurosci 1:4.
Kim MJ, Dunah AW, Wang YT, Sheng M (2005) Differential Roles of NR2A- and NR2B-
Containing NMDA Receptors in Ras-ERK Signaling and AMPA Receptor
Trafficking. Neuron 46:745–760.
Kiss JP, Szasz BK, Fodor L, Mike A, Lenkey N, Kurkó D, Nagy J, Vizi ES (2012) GluN2B-
containing NMDA receptors as possible targets for the neuroprotective and
antidepressant effects of fluoxetine. Neurochem Int 60:170–176.
Kleppe IC, Robinson HP (1999) Determining the Activation Time Course of Synaptic
AMPA Receptors from Openings of Colocalized NMDA Receptors. Biophys J
Lardi-Studler B, Smolinsky B, Petitjean CM, Koenig F, Sidler C, Meier JC, Fritschy JM,
Schwarz G (2007) Vertebrate-specific sequences in the gephyrin E-domain
regulate cytosolic aggregation and postsynaptic clustering. J Cell Sci 120:1371–
Laurie DJ, Wisden W, Seeburg PH (1992) The distribution of thirteen GABAA receptor
subunit mRNAs in the rat brain. III. Embryonic and postnatal development. J
Neurosci 12:4151–4172.
Levelt CN, Hübener M (2012) Critical-Period Plasticity in the Visual Cortex. Annu Rev
Neurosci 35:309–330.
Levi DM, Li RW (2009) Perceptual learning as a potential treatment for amblyopia: A
mini-review. Vision Res 49:2535–2549.
Montey KL, Eaton NC, Quinlan EM (2013) Repetitive visual stimulation enhances
recovery from severe amblyopia. Learn Mem 20:311–317.
Montey KL, Quinlan EM (2011) Recovery from chronic monocular deprivation
following reactivation of thalamocortical plasticity by dark exposure. Nat
Morishita H, Miwa JM, Heintz N, Hensch TK (2010) Lynx1, a Cholinergic Brake, Limits
Plasticity in Adult Visual Cortex. Science 330:1238–1240.
Murphy KM, Balsor J, Beshara S, Siu C, Pinto JGA (2014) A high-throughput semi-
automated preparation for filtered synaptoneurosomes. J Neurosci Methods
Philpot BD, Cho KKA, Bear MF (2007) Obligatory Role of NR2A for Metaplasticity in
Visual Cortex. Neuron 53:495–502.
Pinto JGA, Jones DG, Murphy KM (2013) Comparing development of synaptic proteins
in rat visual, somatosensory, and frontal cortex. Front Neural Circuits 7:97.
Pinto JGA, Jones DG, Williams CK, Murphy KM (2015) Characterizing synaptic protein
development in human visual cortex enables alignment of synaptic age with rat
visual cortex. Front Neural Circuits 9:3.
Pizzorusso T, Medini P, Berardi N, Chierzi S, Fawcett JW, Maffei L (2002) Reactivation
of Ocular Dominance Plasticity in the Adult Visual Cortex. Science 298:1248–
Prange O, Wong TP, Gerrow K, Wang YT, El-Husseini A (2004) A balance between
excitatory and inhibitory synapses is controlled by PSD-95 and neuroligin. Proc
Natl Acad Sci USA 101:13915–13920.
Quinlan EM, Olstein DH, Bear MF (1999) Bidirectional, experience-dependent
regulation of N-methyl-d-aspartate receptor subunit composition in the rat visual
cortex during postnatal development. Proc Natl Acad Sci USA 96:12876–12880.
Robinson RT (2002) Fluoxetine Increases GABAA Receptor Activity through a Novel
Modulatory Site. J Pharmacol Exp Ther 304:978–984.
Ruiz-Perera L, Muniz M, Vierci G, Bornia N, Baroncelli L, Sale A, Rossi FM (2015)
Fluoxetine increases plasticity and modulates the proteomic profile in the adult
mouse visual cortex. Sci Rep 5:12517 Available at:
Sale A, Vetencourt JFM, Medini P, Cenni MC, Baroncelli L, De Pasquale R, Maffei L
(2007) Environmental enrichment in adulthood promotes amblyopia recovery
through a reduction of intracortical inhibition. Nat Neurosci 10:679–681.
Sawtell NB, Frenkel MY, Philpot BD, Nakazawa K, Tonegawa S, Bear MF (2003) NMDA
Receptor-Dependent Ocular Dominance Plasticity in Adult Visual Cortex.
Neuron 38:977–985.
Sohal VS, Zhang F, Yizhar O, Deisseroth K (2009) Parvalbumin neurons and gamma
rhythms enhance cortical circuit performance. Nature 459:698–702.
Stocca G, Vicini S (1998) Increased contribution of NR2A subunit to synaptic NMDA
receptors in developing rat cortical neurons. J Phys 507:13–24.
Szasz BK, Mike A, Karoly R, Gerevich Z, Illes P, Vizi ES, Kiss JP (2007) Direct Inhibitory
Effect of Fluoxetine on N-Methyl-D-Aspartate Receptors in the Central Nervous
System. Biol Psychiat 62:1303–1309.
Tiraboschi E, Guirado R, Greco D, Auvinen P, Maya-Vetencourt JF, Maffei L, Castrén E
(2013) Gene Expression Patterns Underlying the Reinstatement of Plasticity in
the Adult Visual System. Neural Plast 2013:605079–605079.
Tsirlin I, Colpa L, Goltz HC, Wong AMF (2015) Behavioral Training as New Treatment
for Adult Amblyopia: A Meta-Analysis and Systematic ReviewMeta-Analysis of
Behavioral Training for Amblyopia. Invest Ophthalmol Vis Sci 56:4061–4075.
Vetencourt J, Sale A, Viegi A, Baroncelli L (2008) The antidepressant fluoxetine restores
plasticity in the adult visual cortex. Science 320:385–388.
Vetencourt J, Tiraboschi E, Spolidoro M, Castrén E, Maffei L (2011) Serotonin triggers a
transient epigenetic mechanism that reinstates adult visual cortex plasticity in
rats. Eur J Neurosci 33:49–57.
Wang C-C, Held RG, Chang S-C, Yang L, Delpire E, Ghosh A, Hall BJ (2011) A Critical
Role for GluN2B-Containing NMDA Receptors in Cortical Development and
Function. Neuron 72:789–805.
Williams K, Balsor JL, Beshara S, Beston BR, Jones DG, Murphy KM (2015) Experience-
dependent central vision deficits: Neurobiology and visual acuity. Vision Res
Wojcik SM, Katsurabayashi S, Guillemin I, Friauf E, Rosenmund C, Brose N, Rhee J-S
(2006) A Shared Vesicular Carrier Allows Synaptic Corelease of GABA and
Glycine. Neuron 50:575–587.
Wojcik SM, Rhee JS, Herzog E, Sigler A, Jahn R, Takamori S, Brose N, Rosenmund C
(2004) An essential role for vesicular glutamate transporter 1 (VGLUT1) in
postnatal development and control of quantal size. Proc Natl Acad Sci USA
101:7158–7163.
Wu GY, Malinow R, Cline HT (1996) Maturation of a central glutamatergic synapse.
Science 274:972.
Fig 1. Pre- and post-synaptic proteins in ipsilateral V1. In V1 ipsilateral to the
deprived eye, there was no effect of experimental condition on the expression of
Synapsin (A), Synaptophysin (B), PSD-95 (C), or Gephyrin (D) (*p < 0.05; **p < 0.01; ***p
< 0.001; ****p < 0.0001).
Fig 2. Presynaptic vesicle cycling and transporter proteins. In contralateral V1,
Synapsin (A) was not affected by experimental condition. For Synaptophysin (B)
fluoxetine alone had no effect, MD alone caused a loss of expression, but combining
fluoxetine with MD prevented the MD-induced loss. For VGLUT1 (C) fluoxetine alone
or with MD caused a loss of expression, but MD alone increased expression. VGAT (D)
was not affected by experimental condition. (*p < 0.05; **p < 0.01; ***p < 0.001; ****p <
Fig 3. Postsynaptic receptor scaffolding proteins and subunits. In contralateral V1,
PSD-95 (A) and Gephyrin (B) had a similar pattern of changes: fluoxetine alone had no
effect, MD alone caused a loss of expression, but combining fluoxetine with MD
prevented the MD-induced loss and caused super-compensation above normal levels.
GluN1 (C) was reduced by fluoxetine regardless of visual experience, while MD alone
caused an increase. GluA2 (D) was unaffected by fluoxetine alone, MD caused an
increase, but combing fluoxetine with MD caused a decrease. GluN2B (E) was reduced
by fluoxetine regardless of visual experience, while MD had no effect. GluN2A (F)
expression of each experimental group was not different from normal animals, but the
MDed group had higher expression than either fluoxetine alone or fluoxetine combined
with MD. GABAAα3 (G) was unaffected by fluoxetine alone, MD caused an increase,
but combing fluoxetine with MD prevented the MD-induced increase. GABAAα1 (H)
was increased by fluoxetine regardless of visual experience, while MD alone had no
effect. (*p < 0.05; **p < 0.01; ***p < 0.001; ****p < 0.0001).
Fig 4. Postsynaptic receptor subunit balances. Neither fluoxetine, MD, nor fluoxetine
combined with MD affected the relative abundance of GluN1-containing NMDARs and
GluA2-containing AMPARs in contralateral V1 (A). Fluoxetine shifted the relative
abundance of NMDAR subunits in favour of the more mature GluN2A subunit,
regardless of visual experience. MD caused a shift in favour of the more immature
GluN2B (B). Fluoxetine shifted the relative abundance of GABAAR subunits in favour
of the more mature α1 subunit, regardless of visual experience. MD caused a shift in
favour of the more α3 subunit (C). (*p < 0.05; **p < 0.01; ***p < 0.001; ****p < 0.0001).
Fig 5. Pre- and Post-synaptic E/I balance. Presynaptic Index in contralateral V1 (A):
(VGLUT1-VGAT)/(VGLUT1+VGAT). Postsynaptic Index in contralateral V1 (B): (PSD-
95-Gephyrin)/(PSD-95+Gephyrin). We found strikingly similar patters in the pre- and
post-synaptic indexes of E/I synapses. Fluoxetine caused a slight shift towards
inhibition in the presynaptic index and had no effect on the postsynaptic index. MD
caused a strong shift to excitatory markers. Combining fluoxetine and MD kept the
balance at normal levels. (*p < 0.05; **p < 0.01; ***p < 0.001; ****p < 0.0001).
Table 1. Statistical table. Statistical comparisons were made by using Bootstrapping
and Monte Carlo Simulations to generate expected 95% confidence intervals for each
group being tested.
interval vs Normal
interval vs 1wk MD
Fluoxetine + 1wk
V1 Ipsi Synapsin -
Bootstrapping + 0.8112-1.1888
Monte Carlo Simulation
V1 Ipsi Synapsin-
Bootstrapping + 0.8388-1.1612
Monte Carlo Simulation
V1 Ipsi Synapsin -
Bootstrapping + 0.8094-1.1906
Monte Carlo Simulation
V1 Ipsi Synapsin -
Bootstrapping + 0.8378-1.1622
Fluoxetine + 1wk MD
Monte Carlo Simulation
Bootstrapping + 0.8812-1.1188
Bootstrapping + 0.8957-1.1043
Bootstrapping + 0.8783-1.1217
Synaptophysin - 1wk
Bootstrapping + 0.8977-1.1023
Fluoxetine + 1wk MD
V1 Ipsi PSD-95 -
Bootstrapping + 0.6143-1.3857
Monte Carlo Simulation
V1 Ipsi PSD-95 -
Bootstrapping + 0.6678-1.3322
Monte Carlo Simulation
V1 Ipsi PSD-95 - 1wk Normal
Bootstrapping + 0.6117-1.3883
Monte Carlo Simulation
V1 Ipsi PSD-95 -
Bootstrapping + 0.6678-1.3322
Fluoxetine + 1wk MD
Monte Carlo Simulation
V1 Ipsi Gephyrin -
Bootstrapping + 0.7124-1.2876
Monte Carlo Simulation
V1 Ipsi Gephyrin -
Bootstrapping + 0.7491-1.2509
Monte Carlo Simulation
V1 Ipsi Gephyrin -
Bootstrapping + 0.7048-1.2952
Monte Carlo Simulation
V1 Ipsi Gephyrin -
Bootstrapping + 0.7533-1.2467
Fluoxetine + 1wk MD
Monte Carlo Simulation
V1 Contra Synapsin- Normal
Bootstrapping + 0.8105-1.1895
Monte Carlo Simulation
interval vs Normal
interval vs 1wk MD
Fluoxetine + 1wk
V1 Ipsi Synapsin -
Bootstrapping + 0.8112-1.1888
Monte Carlo Simulation
V1 Ipsi Synapsin-
Bootstrapping + 0.8388-1.1612
Monte Carlo Simulation
V1 Ipsi Synapsin -
Bootstrapping + 0.8094-1.1906
Monte Carlo Simulation
V1 Ipsi Synapsin -
Bootstrapping + 0.8378-1.1622
Fluoxetine + 1wk MD
Monte Carlo Simulation
V1 Contra Synapsin- Normal
Bootstrapping + 0.8384-1.1616
Monte Carlo Simulation
V1 Contra Synapsin - Normal
Bootstrapping + 0.8138-1.1862
Monte Carlo Simulation
V1 Contra Synapsin - Normal
Bootstrapping + 0.8381-1.1619
Fluoxetine + 1wk MD
Monte Carlo Simulation
Bootstrapping + 0.8801-1.1199
Bootstrapping + 0.8777-1.1223
Bootstrapping + 0.8959-1.1041
Synaptophysin - 1wk
Bootstrapping + 0.8930-1.1070
Fluoxetine + 1wk MD
V1 Contra VGLUT1 - Normal
Bootstrapping + 0.8693-1.1307
Monte Carlo Simulation
V1 Contra VGLUT1 - Normal
Bootstrapping + 0.8872-1.1128
Monte Carlo Simulation
V1 Contra VGLUT1 - Normal
Bootstrapping + 0.8685-1.1315
Monte Carlo Simulation
V1 Contra VGLUT1 - Normal
Bootstrapping + 0.8876-1.1124
Fluoxetine + 1wk MD
Monte Carlo Simulation
V1 Contra VGAT -
Bootstrapping + 0.6458-1.3542
Monte Carlo Simulation
V1 Contra VGAT -
Bootstrapping + 0.6993-1.3007
Monte Carlo Simulation
V1 Contra VGAT -
Bootstrapping + 0.6512-1.3488
Monte Carlo Simulation
interval vs Normal
interval vs 1wk MD
Fluoxetine + 1wk
V1 Ipsi Synapsin -
Bootstrapping + 0.8112-1.1888
Monte Carlo Simulation
V1 Ipsi Synapsin-
Bootstrapping + 0.8388-1.1612
Monte Carlo Simulation
V1 Ipsi Synapsin -
Bootstrapping + 0.8094-1.1906
Monte Carlo Simulation
V1 Ipsi Synapsin -
Bootstrapping + 0.8378-1.1622
Fluoxetine + 1wk MD
Monte Carlo Simulation
V1 Contra VGAT -
Bootstrapping + 0.6956-1.3044
Fluoxetine + 1wk MD
Monte Carlo Simulation
V1 Contra PSD-95 - Normal
Bootstrapping + 0.6038-1.3962
Monte Carlo Simulation
V1 Contra PSD-95 - Normal
Bootstrapping + 0.6683-1.3317
Monte Carlo Simulation
V1 Contra PSD-95 - Normal
Bootstrapping + 0.6097-1.3903
Monte Carlo Simulation
V1 Contra PSD-95 - Normal
Bootstrapping + 0.6679-1.3321
Fluoxetine + 1wk MD
Monte Carlo Simulation
V1 Contra Gephyrin - Normal
Bootstrapping + 0.7050-1.2950
Monte Carlo Simulation
V1 Contra Gephyrin - Normal
Bootstrapping + 0.7480-1.2520
Monte Carlo Simulation
V1 Contra Gephyrin - Normal
Bootstrapping + 0.7089-1.2911
Monte Carlo Simulation
V1 Contra Gephyrin - Normal
Bootstrapping + 0.7515-1.2485
Fluoxetine + 1wk MD
Monte Carlo Simulation
V1 Contra GluN1 -
Bootstrapping + 0.8909-1.1092
Monte Carlo Simulation
V1 Contra GluN1 -
Bootstrapping + 0.9037-1.0963
Monte Carlo Simulation
V1 Contra GluN1 -
Bootstrapping + 0.8910-1.1090
Monte Carlo Simulation
V1 Contra GluN1 -
Bootstrapping + 0.9053-1.0947
Fluoxetine + 1wk MD
Monte Carlo Simulation
V1 Contra GluA2 -
Bootstrapping + 0.8632-1.1368
Monte Carlo Simulation
interval vs Normal
interval vs 1wk MD
Fluoxetine + 1wk
V1 Ipsi Synapsin -
Bootstrapping + 0.8112-1.1888
Monte Carlo Simulation
V1 Ipsi Synapsin-
Bootstrapping + 0.8388-1.1612
Monte Carlo Simulation
V1 Ipsi Synapsin -
Bootstrapping + 0.8094-1.1906
Monte Carlo Simulation
V1 Ipsi Synapsin -
Bootstrapping + 0.8378-1.1622
Fluoxetine + 1wk MD
Monte Carlo Simulation
V1 Contra GluA2 -
Bootstrapping + 0.8824-1.1176
Monte Carlo Simulation
V1 Contra GluA2 -
Bootstrapping + 0.8605-1.1395
Monte Carlo Simulation
V1 Contra GluA2 -
Bootstrapping + 0.8790-1.1210
Fluoxetine + 1wk MD
Monte Carlo Simulation
V1 Contra GluN2A - Normal
Bootstrapping + 0.7104-1.2896
Monte Carlo Simulation
V1 Contra GluN2A - Normal
Bootstrapping + 0.7063-1.2937
Monte Carlo Simulation
V1 Contra GluN2A - Normal
Bootstrapping + 0.7430-1.2569
Monte Carlo Simulation
V1 Contra GluN2A - Normal
Bootstrapping + 0.7418-1.2582
Fluoxetine + 1wk MD
Monte Carlo Simulation
V1 Contra GluN2B - Normal
Bootstrapping + 0.7772-1.2228
Monte Carlo Simulation
V1 Contra GluN2B - Normal
Bootstrapping + 0.7812-1.2188
Monte Carlo Simulation
V1 Contra GluN2B - Normal
Bootstrapping + 0.8074-1.1926
Monte Carlo Simulation
V1 Contra GluN2B - Normal
Bootstrapping + 0.8120-1.1880
Fluoxetine + 1wk MD
Monte Carlo Simulation
V1 Contra GABAA3 Normal
Bootstrapping + 0.8712-1.1288
Monte Carlo Simulation
V1 Contra GABAA3 Normal
Bootstrapping + 0.8908-1.1092
Monte Carlo Simulation
V1 Contra GABAA3 Normal
Bootstrapping + 0.8729-1.1271
Monte Carlo Simulation
interval vs Normal
interval vs 1wk MD
Fluoxetine + 1wk
V1 Ipsi Synapsin -
Bootstrapping + 0.8112-1.1888
Monte Carlo Simulation
V1 Ipsi Synapsin-
Bootstrapping + 0.8388-1.1612
Monte Carlo Simulation
V1 Ipsi Synapsin -
Bootstrapping + 0.8094-1.1906
Monte Carlo Simulation
V1 Ipsi Synapsin -
Bootstrapping + 0.8378-1.1622
Fluoxetine + 1wk MD
Monte Carlo Simulation
V1 Contra GABAA3 Normal
Bootstrapping + 0.8894-1.1106
- Fluoxetine + 1wk
V1 Contra GABAA1 Normal
Bootstrapping + 0.8751-1.1249
Monte Carlo Simulation
V1 Contra GABAA1 Normal
Bootstrapping + 0.8898-1.1102
Monte Carlo Simulation
V1 Contra GABAA1 Normal
Bootstrapping + 0.8713-1.1287
Monte Carlo Simulation
V1 Contra GABAA1 Normal
Bootstrapping + 0.8883-1.1117
- Fluoxetine + 1wk
Bootstrapping + -0.0675-0.0603
Bootstrapping + -0.0595-0.0523
Bootstrapping + -0.0675-0.0603
GluA2:GluN1 - 1wk
Bootstrapping + -0.0596-0.0525
Fluoxetine + 1wk MD
Bootstrapping + -0.1879-0.0107
Bootstrapping + -0.1755-(-0.0018) -0.0553-0.0735
Bootstrapping + -0.1862-0.0090
Bootstrapping + -0.1738-(-0.0034) -0.0569-0.0752
Fluoxetine + 1wk MD
Bootstrapping + -0.1582-0.0079
-0.2900-(-0.0325) -0.1058-0.1577
interval vs Normal
interval vs 1wk MD
Fluoxetine + 1wk
V1 Ipsi Synapsin -
Bootstrapping + 0.8112-1.1888
Monte Carlo Simulation
V1 Ipsi Synapsin-
Bootstrapping + 0.8388-1.1612
Monte Carlo Simulation
V1 Ipsi Synapsin -
Bootstrapping + 0.8094-1.1906
Monte Carlo Simulation
V1 Ipsi Synapsin -
Bootstrapping + 0.8378-1.1622
Fluoxetine + 1wk MD
Monte Carlo Simulation
Bootstrapping + -0.1463-(-0.0039) -0.0619-0.1650
-0.2744-(-0.0481) -0.0873-0.1392
Bootstrapping + -0.1594-0.0092
-0.2919-(-0.0306) -0.1062-0.1582
Bootstrapping + -0.1472-(-0.0031) -0.0610-0.1641
-0.2729-(-0.0496) -0.0866-0.1385
Fluoxetine + 1wk MD
Bootstrapping + -0.0981-0.1807
Presynaptic E/I -
Bootstrapping + -0.0809-0.1635
Presynaptic E/I -
Bootstrapping + -0.0983-0.1808
Presynaptic E/I - 1wk
Bootstrapping + -0.0770-0.1595
Presynaptic E/I -
Fluoxetine + 1wk MD
Bootstrapping + -0.1202-0.1499
Postsynaptic E/I -
Bootstrapping + -0.0999-0.1295
Postsynaptic E/I -
Bootstrapping + -0.1199-0.1495
Postsynaptic E/I -
Bootstrapping + -0.1008-0.1304
Postsynaptic E/I -
Fluoxetine + 1wk MD
Source: http://eneuro.org/content/eneuro/early/2015/12/24/ENEURO.0126-15.2015.full.pdf
Food intake and social habits in male patients and its relationship to intracytoplasmic sperm injection outcomesDaniela Paes de Almeida Ferreira Braga, D.V.M., M.Sc.,a,b Gabriela Halpern, M.Sc.,a Rita de C assia S. Figueira, M.Sc.,a Amanda S. Setti, B.Sc.,b Assumpto Iaconelli Jr., M.D.,a and Edson Borges Jr., M.D., Ph.D.a,b a Fertility-Assisted Fertilization Centre and b Sapientiae Institute-Educational and Research Centre in Assisted Reproduction, S ao Paulo,Brazil
Movimientos anormales Pilar Guerrero, MD Neuróloga infantil Hospital Militar Nueva Granada Universidad Militar Nueva Granada Los desórdenes del movimiento son la mani- vía piramidal, por consiguiente, no se deben festación clínica de síndromes que involucran a una enfermedad cerebrovascular, ni a una no solo movimientos involuntarios anormales enfermedad por sí misma del cordón espinal, en sí, sino compromiso en la realización de un